Physiology of the Optic Nerve
Leonard A. Levin
The optic nerve is not a peripheral nerve but a white matter tract of the central nervous system (CNS), which projects outside the confines of the cranium. There are two optic nerves, each connecting the retina within each globe to target areas within the brain. Because most of human sensory perception is based on vision, the optic nerves, therefore, carry most sensory information into the brain, and thence to the consciousness. Diseases that affect the optic nerve are, therefore, common causes of blindness. Because the CNS does not generally respond to injury by producing new cells or repairing axons, the blindness from optic nerve disease is typically irreversible. This chapter covers the principal aspects of optic nerve anatomy, physiology, and response to pathologic changes in the context of clinical disease. Wherever possible, references to review articles are used, as well as primary sources of special interest for those interested in more detailed knowledge about particular topics.
TOPOGRAPHIC ANATOMY
RETINAL GANGLION CELL AXONS WITHIN THE NERVE FIBER LAYER
The optic nerve begins with the retinal ganglion cell, which is located in the innermost layer of the retina, within in the ganglion cell layer. Retinal ganglion cells receive input from bipolar cells and amacrine cells and project their axon toward the vitreous; then the axon makes an approximately 90° turn and projects toward the optic nerve head in the nerve fiber layer (Fig. 1). The fibers in the temporal part of the retina (corresponding to the nasal visual field) course away from the fovea, and then once in the nasal retina the fibers turn back toward the optic disc, entering in the superior and inferior portions of the disc. The retinal ganglion cell axons arising from retinal ganglion cells in the nasal retina project more directly to the disc, as follows: The fibers from the nasal half of the macula, forming the papillomacular bundle (or more properly, the maculopapillary bundle), enter at the temporal disc, whereas the fibers arising from ganglion cells nasal to the disc enter at the nasal part of the disc.
In addition, there is strict segregation of those fibers arising from retinal ganglion cells located superior to the temporal horizontal meridian (temporal raphe) and those fibers arising from retinal ganglion cells located inferior to the temporal raphe. Because of this segregation, visual field defects corresponding to the injury to the retinal ganglion cell axons typically have stereotyped patterns (e.g., superior or inferior nasal steps, temporal wedges, or the arcuate scotomas). These are called nerve fiber bundle defects and are covered at length in the chapters on glaucoma.
Once at the optic disc, the ganglion cell axons take a 90° turn away from the vitreous toward the brain (Fig. 2). This marks the beginning of the optic nerve, which is depicted in Figure 3. The neuroretinal rim of the disc is the part of the disc that corresponds to the projection of the nerve fibers into the optic nerve. The cup is the part of the disc that does not contain retinal ganglion cell axons. Depending on the size of the disc and whether there is any developmental or acquired injury, the cup-to-disc ratio may range from 0 to 1.0; the former represents a congenitally full disc, and the latter represents a disc that is completely “cupped out,” most commonly as a result of glaucomatous optic neuropathy.
INTRAORBITAL OPTIC NERVE
Although the retinal ganglion cell axon begins in the inner retina and continues along the nerve fiber layer of the retina, the optic nerve is considered to begin at the optic nerve head. There is an approximately 1-mm component of optic nerve within the intrascleral part of the globe and approximately a 30-mm length of optic nerve from the globe to the optic canal.1 The straight-line distance from the back of the globe to the optic canal is much less (the exact amount depending on individual orbital depth); the relative excess optic nerve is necessary for free movement of the globe during eye movements. In some cases excessive proptosis can exhaust the surplus length of optic nerve, resulting in tethering of the globe by the nerve.
The retinotopic (topographic correspondence of optic nerve fibers to retinal location) segregation of retinal ganglion cell axons, particularly the segregation between axons arising from the superior retina and inferior retina, is gradually lost as the axons enter the nerve. There is only moderate retinotopy in the initial segment of the optic nerve.2 The retinotopy decreases distally3,4 and then becomes ordered for eventual nasal decussation near the chiasm.5 Within the orbit, the optic nerve travels within the muscle cone formed by the superior rectus, lateral rectus, inferior rectus, and medial rectus muscles. Tumors within the cone are common sources of compression of the optic nerve, or compressive optic neuropathy. Examples of these tumors include cavernous hemangioma, hemangiopericytoma, fibrous histiocytoma, lymphoma, and schwannoma. In addition, enlargement of the muscles themselves, particularly the inferior rectus and/or the medial rectus in Grave’s (dysthyroid) ophthalmopathy, may also compress the optic nerve.
THE OPTIC CANAL
The optic nerve enters the cranium via the optic canal, a 5- to 12-mm passage that lies immediately superonasal to the superior orbital fissure. The optic canal contains some axons of sympathetic neurons destined for the orbit, as well as the ophthalmic artery. The latter lies immediately inferolateral to the optic nerve and is covered in dura. At the distal end of the canal, there is a half-moon-shaped segment of dura that overhangs the optic nerve superiorly and, thereby, lengthens the canal by a few millimeters. Within the canal, and immediately posterior to the optic canal, meningeal tissue is adjacent to the optic nerve. Benign tumors of the meninges, or meningiomas, are frequent causes of compressive optic neuropathies in these locations. Small tumors within the canal itself, where there is very little free space, may lead to compressive optic neuropathy without a radiographically visible tumor.
INTRACRANIAL OPTIC NERVE AND THE CHIASM
Once the nerve has entered the cranium, there is a highly variable (3 to 18 mm) length of nerve until the chiasm is reached. The length of the chiasm is approximately 8 mm. The intracranial optic nerve and chiasm are immediately above the planum sphenoidale and sella turcica (which contains the pituitary gland). There is approximately 10 mm between the inferior part of the nerve and the superior part of the pituitary. Tumors of the pituitary that are large enough to compress the chiasm may, therefore, cause compressive optic neuropathy.
The nature of the retinotopic segregation changes as the fibers approach the chiasm; the temporal fibers are destined to remain ipsilateral, whereas nasal fibers cross. The ratio of fibers that cross to those that do not cross is approximately 52:48.6 This small difference between the number of crossing and noncrossing fibers is probably not responsible for the relative afferent pupillary defect seen in disorders of the optic tract, in which an afferent pupillary defect is seen contralateral to the injured tract (see also Chapter 34). Instead, some fibers from specialized cells within the retina that are responsible for the pupillary reflex may cross from the temporal retina into the contralateral optic tract.6
THE OPTIC TRACT AND LATERAL GENICULATE NUCLEUS
Although the optic nerve anatomically ends at the chiasm, the retinal ganglion cell axons continue within the optic tract until the lateral geniculate nucleus or other targets (see later discussion and Chapter 34).
OPTIC NERVE AXON COUNTS AND DIMENSIONS
There are approximately 1,000,000 retinal ganglion cells in each retina, with each cell sending a single axon down the optic nerve. Therefore, there are approximately 1,000,000 axons within the optic nerve. Studies in animals suggest that during development approximately 50% to 100% excess retinal ganglion cells are produced, and during development the number of retinal ganglion cells decreases by programmed cell death, in many cases because not all of the axons reach their target regions within the brain. Likewise in human development, excess retinal ganglion cells die, presumably by programmed cell death during development. There is also a large variation between individuals in the number of axons within the optic nerve.
MICROSCOPIC ANATOMY AND CYTOLOGY
AXONS
The most important components of the optic nerve are the axons of the retinal ganglion cells. Although the retinal ganglion cell bodies are situated within the retina, the axons are all located within the optic nerve. There are no other neuronal cell bodies within the optic nerve, thus, it is a pure white matter tract. Although the optic nerve may contain other small nerves, particularly tiny peripheral nerves (branches of the trigeminal system) that carry pain sensation or control vascular tone, most of the optic nerve is composed of the approximately 1,000,000 retinal ganglion cell axons. Optic nerve axons are collected in fascicles, which are separated by pia-derived septa. The mean axon diameter is slightly less than 1 μm.
OLIGODENDROCYTES AND MYELIN
Axonal conduction in the optic nerve depends on the presence of myelin, a fatty multilaminated structure that insulates each axon and greatly increases the speed and efficiency of conduction. This is discussed at length in the section on axonal conduction. The retrolaminar optic nerve is completely myelinated under normal circumstances. Oligodendrocytes are responsible for axonal myelination in the CNS, and there are approximately 20 patches of myelin contributed by each oligodendrocyte. Each oligodendrocyte may share in myelinating several axons, and each axon is myelinated by several oligodendrocytes. Each axon is myelinated with several lamellae of myelin bilayers, with the number of lamellae varying from axon to axon.9 Although oligodendrogliomas occur in other parts of the CNS, neoplasms of oligodendrocytes within the optic nerve are very rare.
Intraretinal ganglion cell axons are not normally myelinated in most species because oligodendrocytes are absent in the retina. It has been hypothesized that the lamina cribrosa prevents migration of those cells, or their precursors, in development. Occasionally, myelination of part of the nerve fiber layer is seen; it is assumed to be secondary to ectopic oligodendrocytes or myelination of intraretinal axons by Schwann cells (as seen in normal cat10 and rat).11
ASTROCYTES
The astrocyte is a major supporting cell of the optic nerve. Like oligodendrocytes, astrocytes are cells of glial lineage. Astrocytes have several functions, the most important are ionic homeostasis and serving as an energy source. Astrocytes are highly efficient at transporting potassium, and increases in the level of extracellular potassium as a result of repolarization are buffered by the presence of astrocytes. They also may serve as a glycogen storehouse, take up and inactivate neurotransmitters, aid in immune responses, and developmentally regulate axonal extension from the retina to the lateral geniculate nucleus.
Astrocytes and their processes not only make up large portions of the optic nerve parenchyma in positions adjacent to neurons and vessels but also are present wherever neuroectodermal structures are adjacent to connective tissues, such as the pial septa, the adventitia of the central retinal artery and vein, and the pia in a layer named the glial mantle of Fuchs.12
Astrocytes, whether from optic nerve or from other CNS structures, may be immunohistochemically identified by the presence of intracellular glial fibrillary acidic protein (GFAP), an intermediate filament protein akin to the cytokeratins of epithelial cells.
Two types of astrocytes are seen in the retina, the Müller cell and the retinal astrocyte. Müller cells are oriented perpendicular to the retinal plane, span it radially, and originate from neuroepithelium during retinal development.13 Retinal astrocytes are found in the nerve fiber layer, codistributed with retinal blood vessels.14 The retinal astrocyte, like that of the optic nerve, is probably a supporting cell for the ganglion cell axon.15
Astrocyte processes are concentrated at the nodes of Ranvier and have end feet in contact with nearby capillaries, presumably to allow transportation of substances between the local circulation and the axons.
The most common intrinsic tumor of the optic nerve is an astrocytoma, or optic nerve glioma. This is usually a low-grade tumor of well-differentiated astrocytic cells that appear hair-like, or “pilocytic.” Pilocytic astrocytomas are usually seen in childhood and have a favorable prognosis. They are also commonly seen in association with neurofibromatosis. Rarely, more malignant neoplasms of astrocytic origin develop in adults and resemble the higher grade astrocytic neoplasms found elsewhere in the CNS. They are usually fatal.
MICROGLIA
Microglia are tissue macrophages found in the CNS. Although their origin was debated for decades, they have been shown experimentally to be of bone marrow origin16 and to share several markers with macrophages.17 They are associated with axon bundles but not necessarily with blood vessels. Microglia share several characteristics of the immune capacities of macrophages. By phagocytosing extracellular material, degradation within intracellular compartments can occur. This may be followed by antigen presentation on the cell surface and subsequent immune system activation.
MENINGES
The optic nerve is covered with three layers of meninges, dura, arachnoid, and pia. The meninges can also be divided up into pachymeninges (dura) and leptomeninges (arachnoid and pia). The outermost meningeal layer is the dura. This is a thick fibrovascular tissue, which is a direct extension of the sclera and which is in immediate contiguity with the periorbita and the dural layer of the lining of the cranial contents. The dura also continues within the optic canal. The arachnoid is the middle meningeal layer. It is a fairly loose, thin, fibrovascular tissue. The innermost meningeal layer is the pia, a tightly adherent layer that is extremely thin. Extensions of the pia continue within the nerve as the pial septa and provide the blood supply to the intraorbital and intracranial optic nerve, as discussed in the section on vasculature. The fibroblastic cells of the pial septa also form a matrix through which the fascicles of ganglion cell axons course.18 In the optic canal, there are numerous trabeculae connecting the dura through the arachnoid to the pia, which reduce the free space of the nerve sheath in this area.19 The pia extends collagenous septa into the optic nerve parenchyma, containing blood vessels that nourish the nerve.
The space between the dura and arachnoid is the subdural space, and the space between the arachnoid and pia is the subarachnoid space. The subdural space around the optic nerve is small and is not in communication with the intracranial subdural space. The subarachnoid space, on the other hand, is in communication with the intracranial subarachnoid space. The optic nerve subarachnoid space ends anteriorly within a blind pouch just before the disc (see Fig. 3). As discussed in the section on increased intracranial pressure, the extension of the subarachnoid space from the brain into the orbit implies that elevated intracranial pressure has the potential to cause compression of the optic nerve via elevation of the hydrostatic pressure.
Although all three layers of meninges are present in the intraorbital and intracanalicular optic nerve, only the pia continues along the intracranial optic nerve. The clinical relevance of this is that a tumor arising from the optic nerve meninges (i.e., an optic nerve sheath meningioma) will normally continue through the optic canal but then extend along the sphenoid bone and not along the course of the intracranial optic nerve. This means that it is extremely rare for an optic nerve sheath tumor to extend toward the chiasm and, thereby, affect the other side. The more common way for optic nerve sheath meningiomas to become bilateral is by directly extending along the sphenoid bone meninges.
Mast Cells
Mast cells, the tissue equivalent of circulating basophils, are responsible for immediate hypersensitivity (allergic) responses. They contain cytoplasmic granules of histamine, serotonin, and other inflammatory and vasoactive compounds. These granules are released when molecules of immunoglobulin E that are bound to high-affinity receptors on the mast cell surface contact particular target antigens. The products of degranulation are responsible for a local acute inflammatory response, with vasodilation and increased permeability of capillaries causing consequent edema. Cytokines released by mast cells may activate local inflammatory cells or may act as chemoattractants to recruit cells from the circulation. Mast cells have been histochemically identified in the meninges of human optic nerve,20 whereas histamine of mast cell origin has been described in rabbit and bovine optic nerve.21
OTHER CELLS
Blood vessels within the optic nerve contain a variety of cell types. These are discussed further in the section on vascular supply to the optic nerve. Fibroblastic cells within the optic nerve are usually extensions of the meninges, and these are discussed in that section. The lamina cribrosa contains specialized fibroblastic cells; this important structure is discussed in Chapter 25.
TARGETS OF RETINAL GANGLION CELL AXONS
There are four main targets of retinal ganglion cells axons contained within the optic nerve: (1) lateral geniculate nucleus, (2) pretectal nucleus, (3) superior colliculus, and (4) suprachiasmatic nucleus. Most axons are unbranched, but studies in many animals,22 including primates23 have demonstrated the existence of axon collaterals, suggesting that the same may be true for human optic nerves. This section discusses each of the synaptic targets of retinal ganglion cells axons.
LATERAL GENICULATE NUCLEUS
The main function of the optic nerve is for vision, and this is primarily subsumed by projections to the lateral geniculate nucleus (discussed in Chapter 34). The primate lateral geniculate nucleus is a six-layered structure, with layers 1 and 2 consisting of large (magnocellular) cells and layers 3, 4, 5, and 6 consisting of small (parvocellular) cells. The magnocellular layers receive input from the parasol retinal ganglion cells, whereas the parvocellular layers receive input from the midget retinal ganglion cells. Layers 2, 3, and 5 receive input from the ipsilateral retina, whereas layers 1, 4, and 6 receive input from the contralateral retina. In addition, there are interlaminar cells forming the koniocellular layers. These cells are smaller than those of the parvocellular layers and receive synaptic input from blue-yellow retinal ganglion cells.24 The first (and only) synapse of most axons within the optic nerve takes place at the lateral geniculate nucleus. These postsynaptic neurons then project to area V1 of the occipital cortex (i.e., the striate cortex). Details of this projection and the functional anatomy of the part of the cerebral cortex that is devoted to vision is discussed in Chapter 34.
PRETECTAL NUCLEI
A second function of the optic nerve is for the afferent portion of the pupillary response.25,26 These fibers project to the pretectal nuclei within the midbrain. Although the exact number is not known in humans, retrograde labeling studies in pigeons27 and rats28 indicate that only a small percentage of retinal ganglion cell axons are responsible for the pupillary reflex. The pretectal projection is bilateral, and, in addition, fibers from each pretectal nucleus project both ipsilaterally and contralaterally to the Edinger-Westphal subnuclei of the third nerve nucleus. The latter then projects parasympathetic axons along the course of the third nerve to the ciliary ganglion within the orbit, where a synapse is made. Postsynaptic parasympathetic fibers continue to the pupilloconstrictor muscle, constriction of which is visible as the pupillary light reflex.
Because of the combined ipsilateral and contralateral projections from the retina to the pretectal nuclei and from the pretectal nuclei to the Edinger-Westphal subnuclei, a light stimulus that causes impulses along either optic nerve will cause equal constriction of both pupils. This is the physiologic basis for detecting an afferent pupillary defect (also called the swinging flashlight test or Marcus-Gunn pupil). If there is a functional difference in the number of optic nerve axons carrying impulses from each retina, then light shined into the eye with fewer axons will cause a lesser amount of (bilaterally symmetric) pupillary constriction than light shined into the other eye. By alternating the light between the two eyes and observing the difference in pupil size dependent on which eye the light is shined into, one can detect which optic nerve is functionally conducting less than the other. This measure is highly correlated with differences in the number of surviving retinal ganglion cells.29
SUPERIOR COLLICULUS
A third set of optic nerve axons project to the superior colliculus, a paired structure of the dorsal midbrain. Although in lower animals (e.g., rodents) most fibers of the optic nerve project to the superior colliculus, only a minority do so in primates, including humans. The function of the superior colliculus is to coordinate retinal and cortical control of saccades and fixation.30
HYPOTHALAMUS
OPTIC NERVE ELECTROPHYSIOLOGY
SYNAPTIC TRANSMISSION
Retinal ganglion cells receive synaptic input from bipolar and amacrine cells. The bipolar inputs are the results of graded potentials; only retinal ganglion cells exclusively use action potentials (amacrine cells have features of both).34 The synapses from bipolar and amacrine cells primarily use glutamate as the major excitatory neurotransmitter. The retinal ganglion cell synapse on its targets, primarily the lateral geniculate nucleus in higher animals, is glutamate. Within the retina the levels of glutamate are controlled by Müller cells, which have glutamate transporters and also contain the enzyme glutamine synthetase, which converts glutamate to the amino acid glutamine.
EXCITOTOXICITY
Retinal ganglion cells are particularly susceptible to high levels of glutamate in the extracellular space. This causes cell death mediated via overexcitation, or excitotoxicity. It has been proposed that excitotoxic retinal ganglion cell death may explain pathologic conditions, such as glaucoma or retinal ischemia, in which an excess of retina ganglion cells die, akin to what occurs with cerebral infarct or other diseases.
AXONAL CONDUCTION
Action Potentials
The primary function of the optic nerve is to carry information from the retina to target areas within the brain. Individual retinal ganglion cell axons transmit information via action potentials, which are all-or-nothing spikes of electrical activity. This is in contrast with intercellular communication within the retina, where graded potentials are used to transmit information. The retinal ganglion cells and their axons are the first neurons in the transmission of visual information from the eye to use action potentials as the mechanism for transmission. With action potentials, the actual amount of voltage change (i.e., depolarization) is the same, whereas the number of impulses per second and the distribution of impulses within various axons is the mechanism by which visual information is carried down the optic nerve. Conduction down individual axons occurs via the same biophysical mechanisms that occur in any myelinated axon (see later section on myelin).
During axonal conduction, depolarization of a section of membrane induces opening of voltage-sensitive sodium channels located within adjacent membrane. These allow much greater amounts of sodium to enter the axon, and the positive sodium ions cause the axon to become more positive (i.e., depolarized). In this case, the potential across the membrane is now weighted far more by the sodium equilibrium potential than by the potassium equilibrium potential. The sodium channels open because they are voltage sensitive, and a relatively small depolarization of the membrane will cause them to open transiently. Increased potential within the axon rapidly affects adjacent sections of the axon and causes sodium channels in these adjacent areas of axonal membrane to likewise become partially depolarized. These then completely open and cause a compete depolarization. This chain of events continues down the axon, resulting in a transmission of action potential.
Repolarization is the return of the axonal membrane potential to the original (negative resting potential) state and is necessary for more than a single action potential to be transmitted down the axon. Repolarization is due to the closure of the voltage-sensitive sodium channels and a transient opening of voltage-sensitive potassium channels. Once the latter occurs, the membrane resting potential is weighted more by the potassium equilibrium potential than by the sodium equilibrium potential. This results in a restoration of membrane potential to the resting state.
In the process of transmission of an action potential there is movement of sodium and potassium ions along their concentration gradients. If action potentials continued indefinitely, the sodium and potassium concentrations would reach equilibrium across the axonal membrane, resulting in loss of their concentration gradients and blocking conduction. Therefore, to re-establish their concentration gradients, there is a relatively slow redistribution process of these ions via the Na+ = K+ = ATPase takes place. This is a highly energy-dependent process and will not happen if axonal metabolism is pathologically disturbed.
Role of Myelin
Myelin adds an additional complexity to axonal conduction. Myelin has two physiologic properties. It increases the resistance and decreases the capacitance of the axon. The decreased capacitance means less sodium ions (positive charge) are needed to enter the axon to depolarize the membrane to a given voltage. The increased resistance means that there is less leakage of charge across the membrane. Together, these properties decrease the amount of ionic flux needed to achieve changes in voltage across the membrane. If conduction can be achieved with less ionic flux, then less energy is needed to maintain ionic homeostasis after conduction, and, therefore, less Na, K-ATPase activity is necessary. Therefore, myelinated conduction is more efficient.
Ionic channels are not distributed uniformly along a myelinated axon. Instead, the channels are segregated into patches located within the small areas where the axon is unmyelinated. These are called nodes of Ranvier (Fig. 4). Conduction in a myelinated axon, therefore, becomes much faster, because the depolarization of the axon at one node leads to depolarization at the next node by the intrinsic conduction within the axon (the “cable properties” of the axon), instead of by sequential opening of channels along the axon. The depolarization, thus, “jumps” from one node to another; this is called saltatory conduction (see Fig. 4).
In cases of acquired loss of myelin, for example in idiopathic optic neuritis, a common inflammatory optic neuropathy, there is abnormal conduction because of the changes in resistive and capacitive properties of the membrane brought about by demyelination. Axonal conduction becomes slowed and in some cases may be blocked (“conduction block”), both of which result in decreased visual function. Even in a completely demyelinated axon, a low density of internodal sodium channels in demyelinated optic nerve may still allow conduction.35 Another phenomenon peculiar to demyelination is worsening of vision with heat or exercise, called Uhthoff’s phenomenon. Increased temperature and exercise are thought to decrease the sodium channel open-time during depolarization, resulting in less charge entering the axon and a decreased likelihood that an adjacent section of demyelinated axon will be able to depolarize enough to cause opening of its own voltage-sensitive sodium channels. This leads to temperature-sensitive conduction block.
AXONAL TRANSPORT
The entire length of the retinal ganglion cell axon must be maintained by transporting proteins and other subcellular constituents several centimeters from the cell body, where the nucleus and virtually all of the protein synthetic machinery resides. These axons are several centimeters long, and, thus, the health of the nerve relies on axonal transport of a multitude of substances over a distance many times the size of the ganglion cell body. The processes underlying axonal transport have been well studied, in the optic nerve and in other nerves, and several important features can be identified.
Axonal transport occurs in two directions, orthograde (away from the cell body and toward the brain) and retrograde (toward the cell body and away from the brain). By injecting radioactive, fluorescent, or enzymatically active macromolecular tracers into either the eye or the terminal fields of the axons, the course and timing of orthograde and retrograde transport can be determined. By analysis of the individual labeled intra-axonal substances, transport can be differentiated into several classes. Depending on the fineness of the separation, there appear to be two to five groups of substances that are axonally transported, each with their own characteristic velocity.
Fast axonal transport carries several subcellular organelles, such as the vesicles of neurotransmitter used in synaptic transmission, as well as other particulate matter, toward the axon terminal.36 Proteins carried by this process are usually membrane-associated.37 This process has been measured at 90 to 350 mm/day in the rat and chick optic nerve.38,39 Fast axonal transport is sensitive to metabolic inhibitors and to agents such as colchicine, suggesting an energy- and microtubule-dependent mechanism for transport, respectively.40 This class of transport continues despite transection of the axon distal to the cell body, suggesting that intra-axonal components are sufficient for the process to occur.
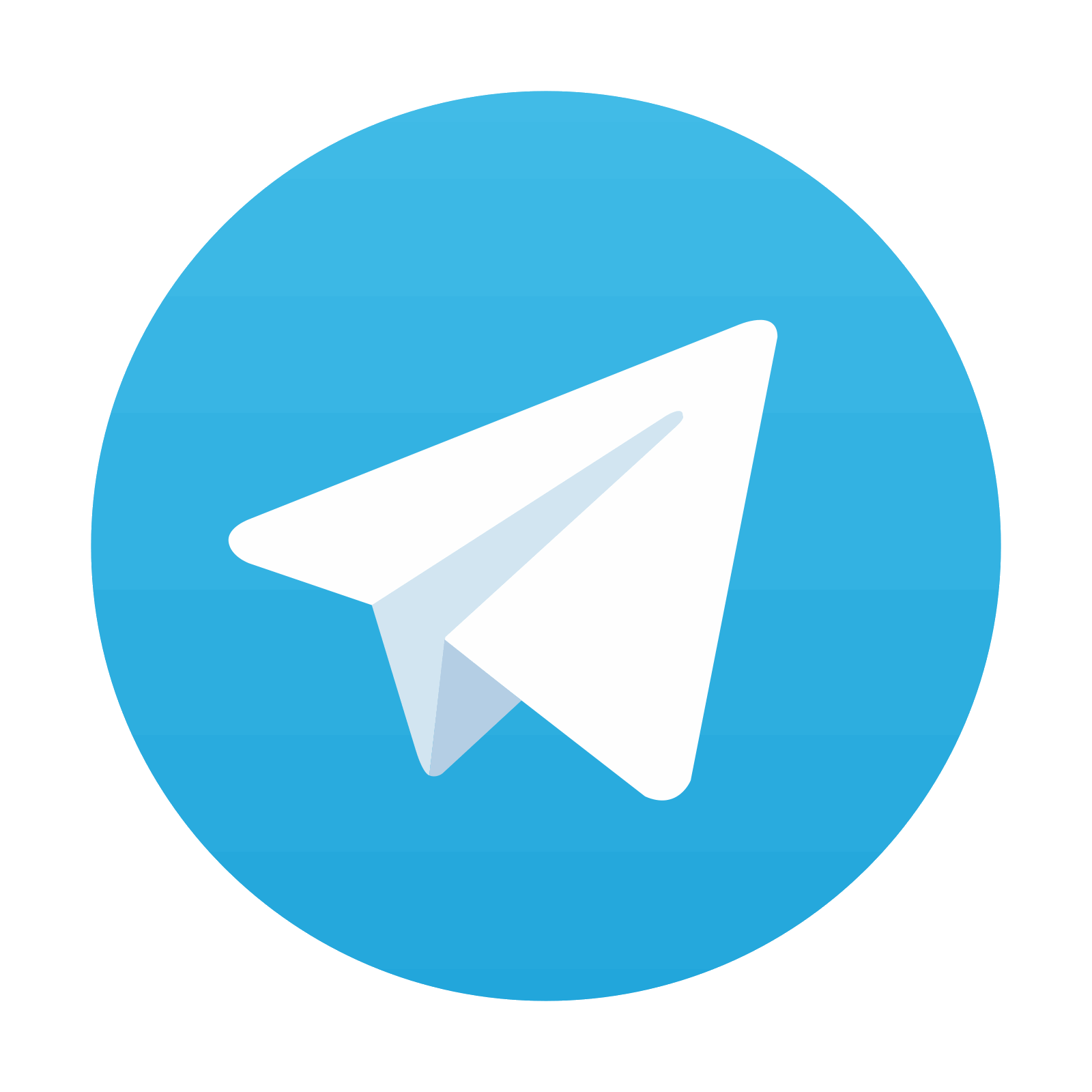
Stay updated, free articles. Join our Telegram channel
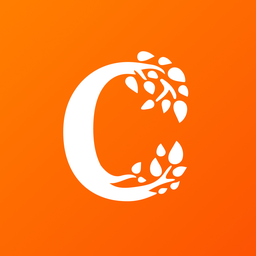
Full access? Get Clinical Tree
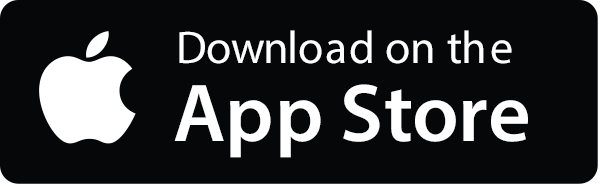
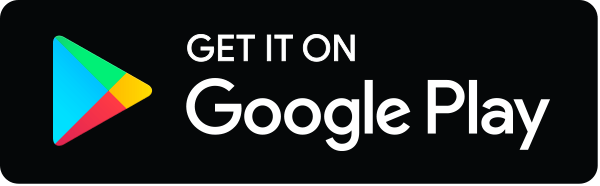