Chapter 24 Laser Revision Stapedectomy
Videos corresponding to this chapter are available online at www.expertconsult.com.
LASER PHYSICS AND PRINCIPLES
Laser energy is derived from the release of energy (photons) occurring when stimulated electrons return to their resting orbital. As proposed by Einstein in 1917, when photons of the appropriate wavelength strike excited atoms, a second additional photon is released as the electron returns to its ground state (Fig. 24-1). In this stimulated emission situation, the photon that is emitted from the excited atom has exactly the same frequency, direction, and phase as the incident photon, providing laser energy that is collimated, coherent, and monochromatic.
Lasers are typically named by the active medium, or the source of atoms that are excited and undergo stimulated emission of photons. The active medium can be a liquid, solid, or gas. Common gas lasers include CO2, argon, and helium-neon. An example of solid state lasers are the neodymium:yttrium-aluminum-garnet (Nd:YAG) and the potassium titanyl phosphate crystal (KTP). The KTP laser is simply a Nd:YAG laser beam that passes through a KTP crystal, which halves the wavelength and doubles the frequency of the laser beam (Table 24-1).
For any laser, the magnitude of the laser-tissue interaction can be regulated by the laser’s power output, the power density at the point of impact, and the energy fluence. Every surgeon who uses a laser should thoroughly understand these fundamental concepts. Power is the time rate at which energy is emitted, and is expressed as watts. The power output is directly adjusted by the control panel on the laser console. Laser energy is delivered through a focusing lens. Power density is a measure of the intensity, or concentration, of the laser beam spot size (Fig. 24-2). It is the ratio of power to surface area of the spot size, and is expressed in terms of watts per square centimeter:
where area of spot size is πr2, and where r = spot size radius in centimeters.
Power density is inversely proportional to the square of the radius of the spot size. Consequently, for any specific power output, changes in the spot size can have a tremendous effect on power density (Fig. 24-3).
As fluence increases, the volume of affected tissue also increases. The thermal energy having an impact on tissue increases dramatically as the time of exposure increases. If power density (W/cm2) is held constant, and exposure time is doubled, the energy delivered is doubled. The thermal effect of the tissue increases significantly, however, because the increase in temperature is continuous. For example, assume the laser power is set at 2 W, the spot size is 0.2 mm2, and exposure time is 0.2 second. Is this the same as delivering two separate impulses at 0.1 second each? Yes and no. Yes, it is the same in terms of energy delivered from the laser. But no, it is not the same in terms of thermal energy imparted to the tissue because during the time between the two separate 0.1 second pulses, no matter how brief, the tissue is cooling (Fig. 24-4). Other factors come into play, such as the absorption characteristics of the damaged tissue in the center of the laser spot and dissipation of heat, but the important concept is that of the increase and decrease of the temperature.
HISTORY OF REVISION STAPEDECTOMY
Background
After the introduction of the stapedectomy procedure by Shea1 in 1958, the surgical treatment of otosclerosis was revolutionized. During the 1960s and 1970s, otologic surgeons performed hundreds of thousands of stapedectomies. An otologic surgeon commonly performed thousands of stapes procedures during his or her peak professional years. The accepted success rate (as defined by closure of the air-bone gap to ≤10 dB) was 90% or greater, with a 1% or less incidence of significant sensorineural hearing loss, including deafness. The most common technique during this time was the total stapedectomy, with removal of the entire stapes footplate. The small fenestra technique began gaining some acceptance, although not universal, in the early 1980s.
As with any surgical procedure, the success rate of stapedectomy was not 100%; with even a small percentage of failures (i.e., air-bone gap closure of >10 dB) in such a large pool of patients, there was a significant number of patients who were candidates for revision stapes procedures. Stapes surgeons soon discovered two important facts: The success rate of revision stapedectomy was not nearly as high as that of primary stapedectomy, and the incidence of significant sensorineural hearing loss, including dead ears, was significantly higher than the incidence associated with primary stapedectomy. Prominent otologists obtained air-bone gap closure within 10 dB in 50% or less of revision cases.2–7 The incidence of significant postoperative sensorineural hearing loss ranged from 3% to 20%, with 14% having profound loss.2,3,6,8
Glasscock2 and Sheehy3 and their associates and Lippy and Schuring8,9 advocated leaving the oval window neomembrane intact and undisturbed, if possible, in revision cases to reduce the risk of severe sensorineural hearing loss, even though it may result in fewer patients with postoperative hearing improvement. Feldman and Schuknecht,4 Pearman and Dawes,10 and Derlacki5 reported opening the neomembrane to identify the vestibule and ensure correct prosthesis placement.
Laser
Concomitant with the realization that revision stapedectomy surgery was not as successful as primary stapedectomy was the introduction of lasers in temporal bone surgery. The use of the laser in temporal bone surgery was the object of experiments in 1967 by Sataloff11 and 1972 by Stahle and colleagues.12 The evolution of laser otologic surgery has been based on a mixture of clinical, experimental animal, and laboratory observations. In 1977, Wilpizeski13 examined argon and CO2 lasers on monkeys by performing myringotomy, ossicular amputation, stapes fenestrations, lysis of stapedial tendon, and crurotomy. He noted damage to the organ of Corti in monkeys after using “excessive power.”
Escudero and associates14 were the first to use a laser in human otologic surgery in 1977. They used the argon laser with a fiberoptic handpiece to tack temporalis fascia to tympanic membrane perforations. In 1979, Perkins15 presented a preliminary report of argon laser stapedotomy with excellent initial results in 11 patients. In 1980, DiBartolomeo and Ellis16 expanded argon laser applications in 30 patients for middle ear and external ear soft tissue and bony problems. In 10 patients, otosclerosis was corrected, including one revision case. In 1983, McGee17 reported on the use of argon laser in more than 500 otologic cases, 100 of which were primary stapedectomies. There were no laser-related complications in his study. In 1989, McGee18 reported an update on 2500 tympanomastoid procedures, of which 510 were primary stapedectomies. By comparing 100 consecutive laser stapedectomies with a previous 139 small fenestra stapedectomies using instruments, McGee found that the laser technique permitted much shorter hospital stay, less vertigo, and excellent hearing results (93% air-bone gap closure ≤10 dB at 6 months). This large study indicated the safety of argon laser use for stapedectomy, and yielded comparable hearing results and less vertigo. This clinical evidence is inconsistent with experimental animal studies, in which temporary changes of cochlear microphonics and saccular perforations were noted.17,19,20 Clinical experience from several centers has illustrated the safe use of lasers in ear surgery; however, arguments and opinions persist regarding the best type of laser.21–25
Comparison of Lasers
The visible-wavelength laser beam can be carried by thin fiberoptic cables, which allow two modes of delivery: a micromanipulator attached to the microscope or a hand-held probe (Fig. 24-5). The hand-held probe provides greater angle of divergence of the laser beam (i.e., rapid deterioration of power density) and must be placed very close to the tissue. This instrument is directly in the operative field and can obstruct a portion of the visual operative field. With the micromanipulator, the angle of the laser beam is less divergent. It does not require an instrument in the field (except a suction for smoke plume removal), but does require the use of a “joystick” control mechanism to direct the beam. The CO2
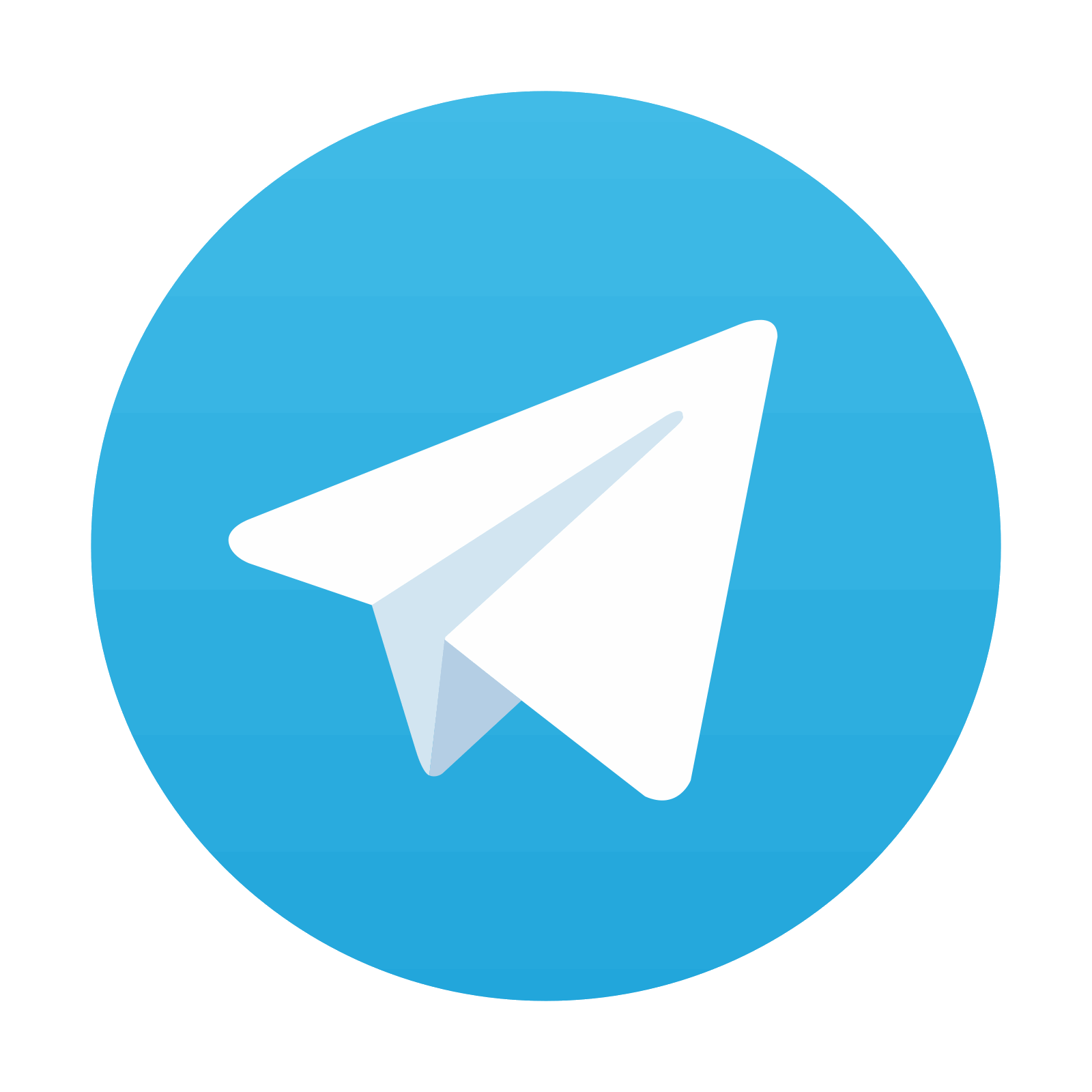
Stay updated, free articles. Join our Telegram channel
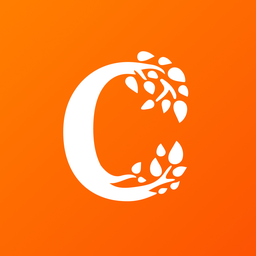
Full access? Get Clinical Tree
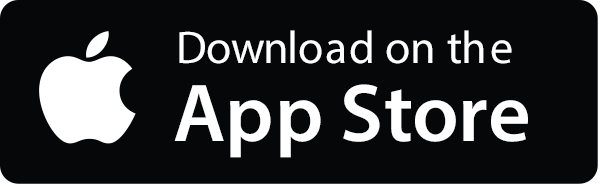
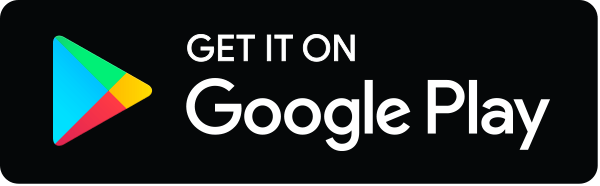