Fig. 10.1
SS-OCT system and examples of imaging interfaces. (a) Photograph of the SS-OCT ophthalmic interface. (b) Schematics of the SS-OCT system along with the different interface designs for (c) anterior segment, full eye length imaging and long-range OCT imaging, (d) retinal imaging, (e) SS-OCM, and (f) endoscopic imaging (micromotor probe). FC fiber coupler, PDB1/PDB2 balanced photodetector, MZI Mach-Zehnder interferometer, SC galvanometric scanners, COIL torque coil, GRIN gradient index lens, MM micromotor
Figure 10.1 shows a schematic for a representative swept source imaging system at 1,050 nm wavelengths. The system uses a transmission geometry for the reference arm because high-quality optical circulators are not available at this wavelength. Several different OCT imaging systems were used to generate the data shown in this chapter. SS-OCT systems can be interfaced to microscopes, handheld imaging probes, as well as catheters and endoscopes. Different interface designs can be developed depending on the application (Fig. 10.1c–f). In the simplest microscope configuration, the interface has galvanometric scanners at the back focal length of a lens to provide telecentric scanning (Fig. 10.1c). This design is used to image the anterior segment and the full eye length as well as to image non-biomedical objects with long-range OCT. Retinal imaging requires an interface with an adapter lens to collimate the incident beam on the eye and relay the beam scanning to pivot at the pupil of the eye (Fig. 10.1d) [30]. For swept source optical coherence microscopy (SS-OCM), the sample arm is interfaced to a scanning confocal microscope (Fig. 10.1e) [31]. The fiber output is collimated and relayed to the objective by a pair of compound lenses. Objectives with 10×, 20×, and 40× magnifications can be used, thereby enabling imaging with different fields of view and transverse resolutions. Another example configuration shown in Fig. 10.1f is a micromotor-based miniature catheter for ultrahigh-speed endoscopic OCT imaging [32]. The OCT beam was focused by a fiber-GRIN lens assembly, reflected by the rotating microprism, and focused outside the catheter sheath. The motor and GRIN lens are mounted inside a metal hypotube, and the entire assembly is covered by a transparent plastic sheath. The rotating optics could be pulled back within the sheath to produce a spiral volumetric scan pattern. The endoscopic configuration operated at 1,310 nm wavelength, and circulators were used in the interferometer for improved light collection efficiency.
In the SS-OCT configuration shown in Fig. 10.1b, light from the sample and reference arms was interfered with a fiber coupler and the signal was detected by a high-speed, dual-balanced InGaAs photodetector receiver PDB1. The photodetector signal was digitized by a high-speed A/D converter. A trigger for starting sweep acquisition was synchronized with the MEMS VCSEL. A second dual-balanced photodiode PDB2 detected interference fringes from an MZI to generate an optical clock signal. When fixed frequency A/D clocking is used, the MZI signal is used to recalibrate the OCT interference fringes, i.e., to resample the OCT signal from constant time interval to evenly sampled frequency or wave number before Fourier transforming to obtain the axial scan. If optical clocking is used, the MZI generates the optical clock signal with a constant frequency or wavenumber interval determined by the MZI delay. Several A/D acquisition boards (digitizers) were used including a 12-bit 500MSPS A/D converter (ATS9350; Alazar Technologies Inc.) and 8-bit card 1GSPS A/D converter (ATS9870; Alazar Technologies Inc.).
Frequency swept lasers are a core technology for SS-OCT. The studies presented in this chapter were performed with two types of swept light sources utilizing MEMS technology: a short cavity laser (Axsun Technologies Inc.) and a vertical cavity surface-emitting laser (VCSEL, Praevium Inc./Thorlabs Inc.). The short cavity laser had few centimeter cavity lengths and was tuned with an MEMS-tunable Fabry-Perot filter. The VCSEL had a micrometer length cavity and was tuned with a movable MEMS cavity mirror. The micrometer cavity length means that only one longitudinal mode is within the laser gain bandwidth. Single mode operation and absence of mode hopping makes the VCSEL coherence length extremely long and significantly reduces parasitic sensitivity roll-off with imaging range. The coherence length of the VCSEL was compared with an MEMS Fabry-Perot tunable short cavity laser by acquiring interference signals from a Mach-Zehnder interferometer with high bandwidth oscilloscope. Figure 10.2 shows sensitivity versus depth, where the total optical path delay is two times the depth. The VCSEL light source coherence length exceeds 1 m (measurement limited by the oscilloscope bandwidth), whereas the commercial MEMS Fabry-Perot tunable short cavity laser has a coherence length of ∼12 mm.
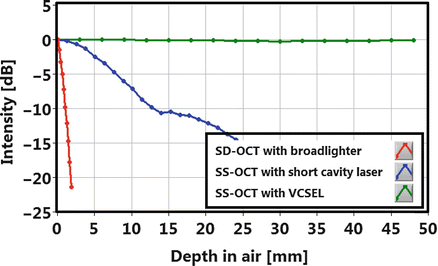
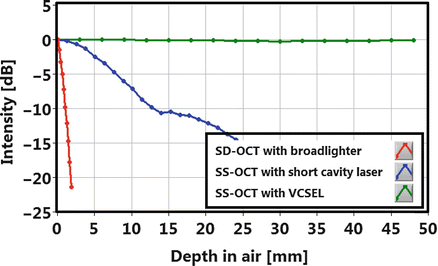
Fig. 10.2
Comparison of the parasitic sensitivity roll-off with depth: MEMS-tunable short cavity laser (sweep rate 100 kHz, tuning range 100 nm), MEMS VCSEL (sweep rate 50 kHz, tuning range 45 nm), and typical high-resolution SD-OCT (810 nm broadband light source)
10.3 High-Speed Imaging with SS-OCT
10.3.1 Imaging Speed in OCT
The imaging speed in OCT is typically specified as the axial scan rate or number of axial scans acquired per unit time. Imaging speed also governs the system sensitivity, i.e., the ability to detect weak signals trades off with increased imaging speed. The imaging speed and the resulting acquisition time is one of the key parameters for ophthalmic imaging as well as many other in vivo imaging applications. With the maturation in OCT technology, systems with higher and higher speeds have become available. The imaging speed in TD-OCT is determined by the periodic mechanical scanning of the reference arm. In practice, TD-OCT instruments are unable to reach speeds higher than ∼2 kHz due to mechanical limitations in the reference arm as well as limitations in detection sensitivity [33]. In SD-OCT, imaging speed is determined by the reading speed of the line-scan camera in the spectrometer. Early SD-OCT instruments operated at 19 kHz axial scan rates, but the development of new line-scan camera technology allowed significant increases in speed so that current SD-OCT systems can operate at more than 100 kHz rates [34]. On the other hand, the imaging speed in SS-OCT is determined by the sweep repetition rate of the swept light source, and modern systems can achieve MHz axial scan rates [18, 26, 32, 35].
Different techniques have been developed to increase OCT imaging speed. The use of multiple beams in the sample arm enabled multiplication of the effective speed, although this approach is complex and requires more sophisticated hardware [18, 22, 36–38]. A parallel solution in SD-OCT is to use a dual-camera system which doubles the acquisition speed [39]. Multiboard acquisition and optical demultiplexing has been used to achieve speeds of 60,000,000 axial scans per second [40]. Using certain swept light sources, it is possible to obtain higher SS-OCT imaging speeds by more efficient usage of the sweep duty cycle. In this approach, imaging speeds can be increased by using both forward and backward sweeps or buffering to combine time-delayed copies of the sweep [16, 22, 41].
10.3.2 High-Speed Retinal SD-OCT and SS-OCT Imaging
The most common application of OCT is retinal imaging. OCT enables imaging the morphology of retinal layers in the fovea and optic disc regions for diagnosis and monitoring therapeutic response in diseases such as age-related macular degeneration, glaucoma, and diabetic retinopathy. Initial retinal TD-OCT instruments had imaging speeds of a few hundred axial scans per second [1, 42, 43]. The introduction of Fourier-domain detection resulted in a sensitivity increase of 50–100 times over previous time-domain technology. These increases in speed enabled dramatic improvements in image quality and retinal coverage, as well as three-dimensional OCT (3-D OCT) imaging. SD-OCT retinal imaging was first demonstrated by Wojtkowski et al. in 2002 [44]. With high-speed line-scan cameras, studies demonstrating SD-OCT at 26,000–29,000 axial scans per second were reported by several groups [45–47]. Ultrahigh SD-OCT imaging speeds of >300,000 axial scans per second were demonstrated by Potsaid et al. in 2008 using advanced CMOS camera technology [34]. Meanwhile, SS-OCT has also undergone similar development. The first SS-OCT retinal instruments operated at imaging speeds of 19,000 axial scans per second [6]. With the advent of new laser technologies, ultrahigh-speed OCT imaging of the retina became possible. Although fundamental laser sweep rates reach 400 kHz, different methods to increase the effective sweep rate were developed including buffering or simultaneous multi-spot imaging. These advances enabled imaging at the rates of several MHz [18, 48]. With these technological improvements, the term “high-speed imaging” was extended to hundreds of thousands of axial scans per second and the term “ultrahigh-speed imaging” referred to speeds which approach ∼1,000,000 axial scans per second [18]. By contrast, current commercial retinal SD-OCT and SS-OCT instruments at 840 nm and 1,050 nm wavelengths have imaging speeds of up to ∼100 kHz.
High acquisition speeds enable 3-D OCT volumetric imaging by raster scanning multiple cross-sectional images. Unlike standard ophthalmic examination techniques such as fundus photography, OCT provides depth-resolved information and enables visualization of 3-D retinal structure. Volumetric data sets provide detailed structural information on the retina that is unavailable with other imaging modalities. The volumetric data sets can be displayed in multiple ways. Figure 10.3 shows SS-OCT retinal imaging with a short cavity laser at 1,050 nm wavelength. Methods of OCT data presentation include 3-D rendering, cross-sectional images, and en face views (OCT projection and C-scan images). Another advantage of 3-D OCT imaging is the ability to extract arbitrary (virtual) cross-sectional images which are precisely registered to retinal fundus features. OCT retinal fundus images can be obtained by summing the entire signal in axial direction. Other types of en face images include projection views that can be generated either by choosing specific depth level or by integrating the signal from selected depth range [49]. The wide variety of data presentation options enhances the diagnostic utility of 3-D OCT imaging in clinical practice and forms a basis for quantitative data analysis.
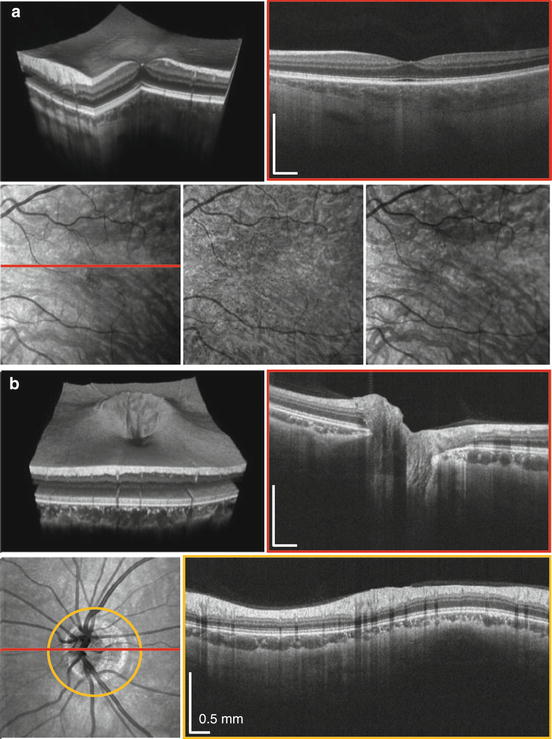
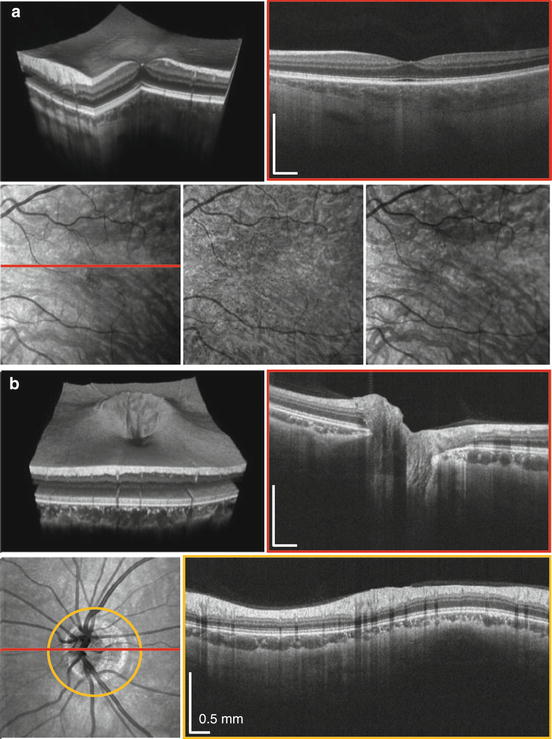
Fig. 10.3
Volumetric SS-OCT retinal imaging at 1,050 nm of the macula (a) and optic nerve head (b) at 1,050 nm wavelength. 3-D data sets enables generation of generating volumetric renderings, OCT fundus images, different cross-sectional images, and C-scans or en face images. Two perpendicularly scanned data sets, each with 700 × 700 axial scans, were acquired at 100 kHz, and an OCT motion correction algorithm was applied to remove motion artifacts
Eye and head motion during acquisition generates artifacts in OCT images. Different approaches have been developed to minimize or compensate motion artifacts, including reducing acquisition times, software-based methods (e.g., OCT registration and motion correction) [50], and hardware techniques (e.g., eye tracking) [51]. Ultrahigh imaging speeds enable rapid acquisition of dense volumetric data to reduce motion artifacts. Figure 10.4a shows 3-D OCT data sets of the optic nerve head of the same subject acquired at three different speeds. Since the lateral scanning density (500 axial scans and 500 B-scans over a 6 × 6 mm2 retina area) was kept constant, different axial scan rates resulted in reduced acquisition times ranging from 2.6 to 0.5 s. Fundus features were used to confirm that corresponding cross-sectional OCT images are extracted from the same position for comparison. These data demonstrate that motion artifacts in the slow scan direction, perpendicular to the raster, are significantly reduced as the imaging speed increases.
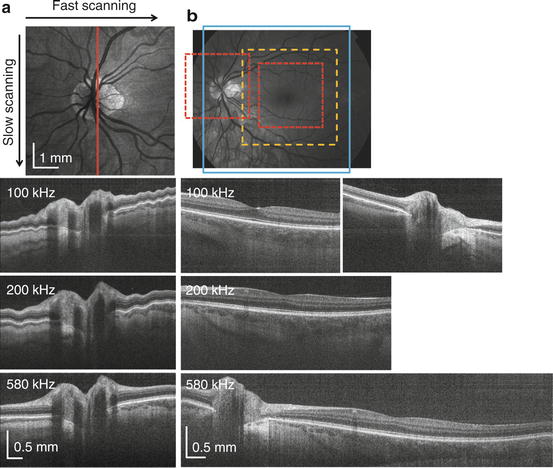
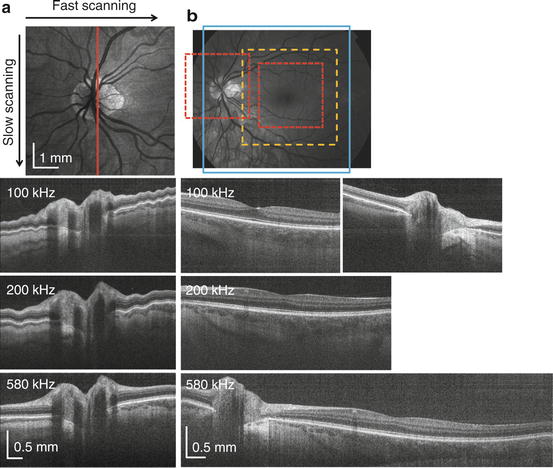
Fig. 10.4
Retinal OCT imaging using SS-OCT with a VCSEL light source. (a) Imaging of the optic nerve head region at different speeds. Extracted central cross sections in the slow scan direction, perpendicular to the raster scan directions, show reduced motion artifacts with increased speed. In each case, the data consisted of 500 × 500 axial scans from a 6 × 6 mm2 area. (b) Impact of the imaging speed on retinal coverage. Red-free fundus photograph indicates scanned areas at different speeds. Selected cross sections from volumetric data sets acquired at 100 kHz, 200 kHz, and 580 kHz. Transverse sampling density and acquisition time are kept constant. Aspect ratios of all cross sections are the same (From Ref. [30])
High-speed OCT imaging of the retina also enables better retinal coverage for a given sampling density. This feature of high-speed imaging is shown in Fig. 10.4b. Volumetric OCT data sets were acquired by raster scanning at axial scan rates of 100 kHz, 200 kHz, and 580 kHz. Fundus views and corresponding cross-sectional images are presented. A measurement time of ∼2 s was used for each volume, consistent with a typical clinically acceptable acquisition time. The data sets comprise of 400 × 400, 600 × 600, and 1,000 × 1,000 axial scans and cover areas of 5 × 5 mm2, 7 × 7 mm2, and 12 × 12 mm2 at the sweep rates of 100 kHz, 200 kHz, and 580 kHz, respectively. Whereas at 100 kHz the scanned area requires separate acquisitions for the central macular region versus the optical nerve head, imaging at 580 kHz enables an almost sixfold increase in scanned area covering both the macular region and optical nerve head in a single scanned area. In this case, a wide-field OCT coverage comparable to standard fundus photography is achieved (Fig. 10.5b compared to Figs. 10.4a and 10.5h), and the sampling density in the transverse direction is high enough to image focal retinal pathologies. Whereas fundus photographs reveal two-dimensional information, OCT volumetric data can be used to display cross-sectional and en face projections of different retinal and choroidal layers. Cross-sectional images in Fig. 10.4 demonstrate the ability to visualize deep choroidal layers, the choroid-scleral interface, and even scleral vasculature due to the high sensitivity and deep image penetration at 1,000 nm wavelengths. Axial summation of the OCT signal intensity from 40 μm thick slices at different depths below the retinal pigment epithelium (RPE) was used to generate projection OCT en face images of the choroid. OCT projection images corresponding to the chriocapillaris, Sattler’s layer, and Haller’s layer can be identified and characterized by choroidal structure and vasculature appearance, as shown in Fig. 10.5 d–g.
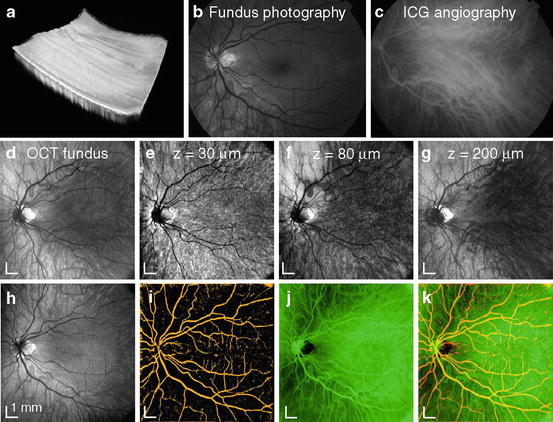
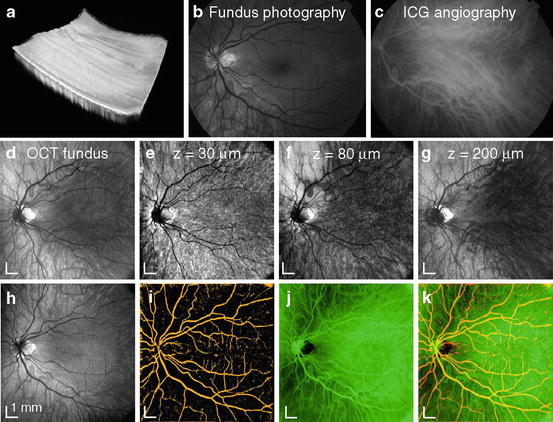
Fig. 10.5
Wide-field choroidal OCT imaging using SS-OCT with a VCSEL light source. (a) Rendering of a volumetric wide-field data set. (b) Red-free fundus photography and (c) indocyanine green (ICG) angiography of the same subject. (d) OCT fundus image. (e) – (g) OCT projection images at different depths below RPE showing choroidal layers and sclera. The OCT signal was integrated from 40 μm thick slices. (h) OCT wide-field fundus image. OCT angiographic images showing: (i) segmented retinal, (j) choroidal vasculature and (k) combined angiographic image. (From Ref. [30])
Standard clinical angiographic modalities require intravenous administration of dyes such as fluorescein or indocyanine green (ICG). However, OCT can be used to visualize vascular networks and generate images analogous to angiography without the need for exogenous contrast agents. This complementary information can be obtained from the same OCT data sets. Figure 10.5 c, h–k show comparisons of ICG angiography and OCT intensity-based retinal and choroidal images. Since retinal vessels generate shadows in OCT cross-sectional images, it is possible to increase contrast in a projection image by summing the intensity from a 50 μm thick layer around the RPE. On the other hand, choroidal vasculature can be visualized by using an inverted gray scale in the projection image of the signal below the RPE. Due to shadowing effects, retinal vessels also appear in the choroidal vasculature en face image. Volumetric OCT data can be used to visualize vascular networks in the eye by a combination of retinal and choroidal projection OCT images. In addition, a variety of Doppler and angiographic OCT methods have been developed that can measure flow using the Doppler phase shift or enhance the contrast of vasculature using phase variance or intensity speckle decorrelation [52–62].
10.3.3 High-Speed Anterior Segment Imaging with SS-OCT
Imaging the anterior segment of the eye requires a combination of long depth range and high imaging speed to obtain clinically useful information. Generally speaking, the challenge of anterior segment OCT imaging comes from the fact that the anterior segment has multiple structures: some of which are anatomically thick, others are heavily pigmented, and one, the cornea, has steep curvature. Additionally, as in other ophthalmic applications, high acquisition speeds are important to minimize motion artifacts.
The first demonstration of anterior segment OCT imaging was performed in 1994 by Izatt et al. [63]. Multiple studies demonstrated time-domain OCT and Fourier-domain OCT for anterior segment imaging at different wavelengths and speeds [11, 22, 64–68]. Challenges encountered in SD-OCT could be addressed by reducing parasitic sensitivity roll-off and improving light penetration into tissue [69, 70]. These requirements can also be fulfilled by SS-OCT technology which offers better performance over SD-OCT at wavelengths longer than the traditional 840 nm used for retinal imaging [4]. Light at wavelengths longer than 840 nm exhibits less scattering and therefore enables deeper imaging [71, 72]. The first SS-OCT systems for anterior segment imaging utilized light sources at 1,300 nm wavelength, and the imaging speed increased from 20 to 200 kHz [11, 66]. Recent demonstrations of anterior segment imaging achieved imaging speeds of 1,600 kHz at 1,310 nm wavelength and 100 kHz at 1,050 nm wavelength [22, 73]. Finally, SD-OCT and SS-OCT systems for simultaneous anterior segment and retinal imaging have also been demonstrated [74, 75].
High-speed and reduced parasitic sensitivity roll-off is important for 3-D imaging of the anterior eye. Figure 10.6a shows a rendering of a volumetric data set covering a 13 × 13 mm2 area of the anterior eye. The volume consists of 500 × 500 axial scans acquired in 2.6 s. The en face image of the anterior segment reveals a rhombus-like polarization artifact from the cornea. Due to the long coherence length of the VCSEL light source, there is virtually no parasitic sensitivity roll-off. This enables direct visualization of the entire anterior segment. The cross-sectional image in Fig. 10.6a shows the cornea, iris, and entire crystalline lens and spans the entire transverse width of the anterior chamber, from limbus to limbus. The crystalline lens is not an optically homogenous structure; the nucleus and cortex can be distinguished. It should be noted that anterior segment OCT images do not display the true shape of the eye unless refraction correction algorithms are used. The refraction-corrected cross-sectional image where the OCT beam propagation direction has been corrected using the interfaces and refractive indices of ocular structures is also shown. The uncorrected image has an artificial posterior displacement of the iris and anterior lens which is produced by refraction of the OCT beam at the air-corneal interface, while the refraction-corrected image displays the iris and anterior lens in their true anatomical positions.
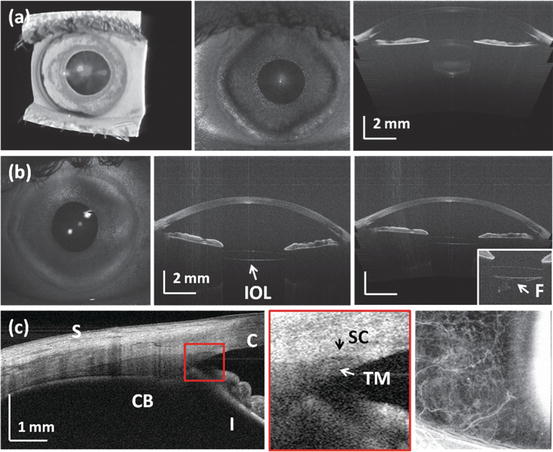
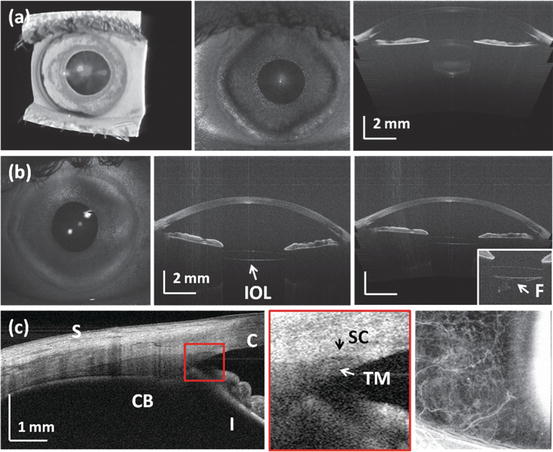
Fig. 10.6
Anterior segment imaging with SS-OCT. (a) Volumetric rendering, en face OCT, and cross section taken from the volumetric OCT data of a healthy subject. (b) En face OCT and cross-sectional images of an eye after cataract surgery and intraocular lens (IOL) implantation (F floaters). (c) Cross section and en face image of the corneoscleral junction with enlargements showing Schlemm’s canal and scleral vasculature (S sclera, CB ciliary body, C cornea, I iris, SC Schlemm’s canal, TM trabecular meshwork)
The same scanning protocol was applied to scan the eye of a subject after cataract surgery. As shown in the en face image in Fig. 10.6b, the intraocular lens (IOL) causes strong reflections in OCT images. Visualization of 3-D data enables identification of regions with floaters behind the posterior IOL surface.
In addition to wide-field OCT, scanning can be performed over specific structures such as the limbus and anterior chamber angle [76]. Figure 10.6c shows corneoscleral imaging of the anterior eye. This does not require long imaging range, and therefore the system was operated at 100 kHz in high-resolution mode, similar to that used for retinal imaging. The volumetric data set consisted of 500 × 500 axial scans and covered a 7 × 7 mm2 area. This dense scan over the anterior chamber angle enabled visualization of the limbal region along with landmarks such as the corneoscleral junction and rich scleral vasculature. Elements of the outflow system such as Schlemm’s canal can also be identified.
After refraction correction of anterior segment OCT data sets, qualitative 3-D structure can be accurately visualized and quantitative information about the shape of ocular structures can be extracted. Figure 10.7 shows example maps of clinically relevant parameters which can be measured from 3-D OCT data. Mapping biometric parameters is important for the diagnosis of ocular diseases as well as in pre-and postoperative assessment of the eye for keratorefractive surgery or IOL implant.
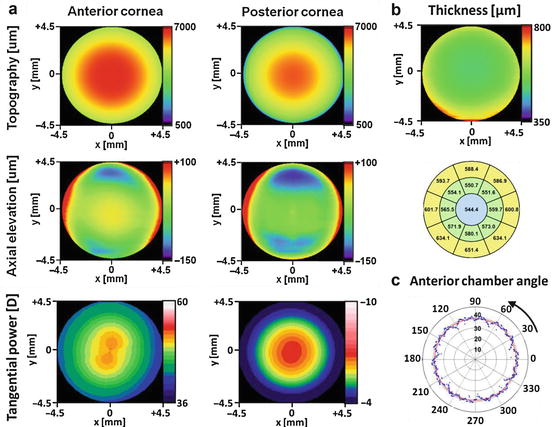
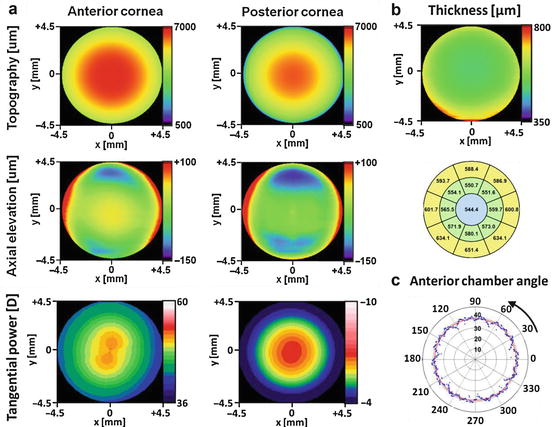
Fig. 10.7
OCT ocular biometry. (a) Topography, elevation, and tangential power (keratometry) maps of both corneal surfaces obtained from 3-D OCT data. (b) Corneal thickness map and corresponding sector map showing average thickness in central (0–3 mm diameter), paracentral (3–6 mm diameter annulus), and pericentral (6–9 mm diameter annulus) region. (c) Polar plot of anterior chamber angle for different meridionals. Mean anterior chamber angle is shown in red
10.3.4 Dynamics of the Anterior Segment of the Eye and 4-D OCT Imaging
Apart from its structural complexity, the eye is also a highly dynamic organ that can adapt to changing conditions and provide the best optical imaging of objects onto the retina. Ultrahigh-speed OCT enables visualization of dynamic processes within the eye [77]. Studies of dynamic processes are an important indicator of ocular functionality and can be performed using rapid, repeated volumetric scanning in time. This demanding scanning mode combines 3-D scanning with time as the fourth dimension and therefore is often described as 4-D imaging. In this subsection, we will discuss applications of high-speed anterior segment imaging for visualizing processes such as tear film breakup and pupillary reflex.
The first example demonstrates measurement of tear film dynamics for tear evaluation and diagnosis of dry eye. The tear film is the first refractive surface for light incident on the eye and plays an important role in the optical quality of the eye. Tear film breakup time is usually measured with fluorescein dye [78]. OCT can enable indirect but noninvasive imaging of tear film dynamics by integrating the detected OCT signal over the lens region where optical shadowing from tear film breakup appears. Figure 10.8a shows the shadows caused by tear film breakup which appear over the lens region in repeated volumetric OCT data sets. A 4-D scan protocol was used with 300 × 300 axial scan volumes over a central 8.5 × 8.5 mm2 area at a rate of ∼0.5 volumes per second for 20 s with an axial scan rate of 50 kHz. The shadowing effect can be observed, and the signal between the anterior and posterior surface of the crystalline lens was used to generate projection OCT images (Fig. 10.8b). Tear film breakup appears as randomly distributed spots in the OCT projection image within the pupil area. Tear film breakup can be determined by the frame-by-frame analysis of the projections and is approximately 12 s in the example shown.
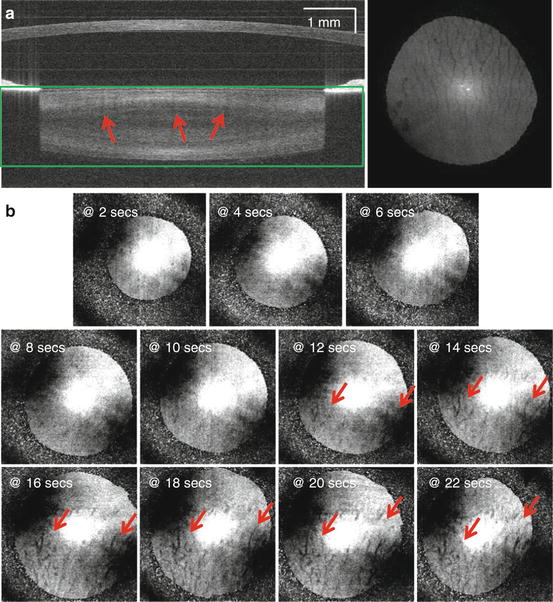
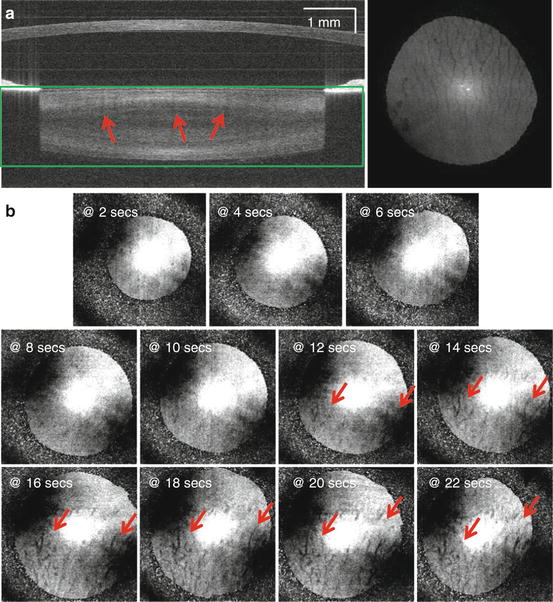
Fig. 10.8
SS-OCT visualization of tear film breakup. (a) Shadowing effect in a cross-sectional image of the anterior segment. The signal from the crystalline lens region can be integrated to generate projection OCT image with a characteristic pattern which shows tear film breakup. (b) Tear film breakup observed in en face images from 4-D OCT data. Tear film breakup is observed to begin at ∼12 s (From Ref. [77])
The iris is a dynamic structure whose configuration changes in response to light and during accommodation. Studying the dynamic response of the pupil to dark-light stimulus may provide a more comprehensive assessment of risks from primary-angle closure development and may help understand the pathophysiology of angle closure glaucoma [79, 80]. Figure 10.9 shows the pupil response to light stimulus from an LED positioned adjacent to the eye. Sequential 150 × 150 axial scan volumes over a 17 × 17 mm2 area were acquired with an ∼8 volumes per second volume rate at an axial scan rate of 200 kHz. The acquisition required ∼5 s and enabled visualization of 3-D changes in the iris as a function of time. The pupillary response to light stimulus can be quantitatively analyzed by measuring the pupil size/area changes. As shown in Fig. 10.9, the pupil area decreased rapidly when light stimulus was applied. In contrast, the time constant of the pupil recovery was longer than the constriction reflex.
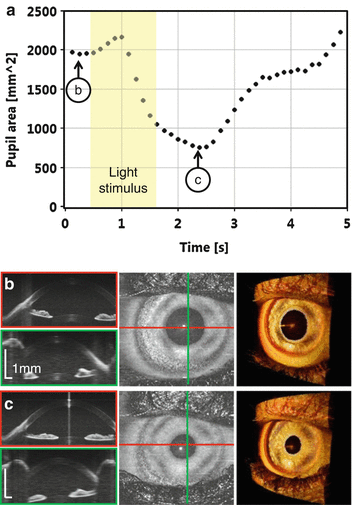
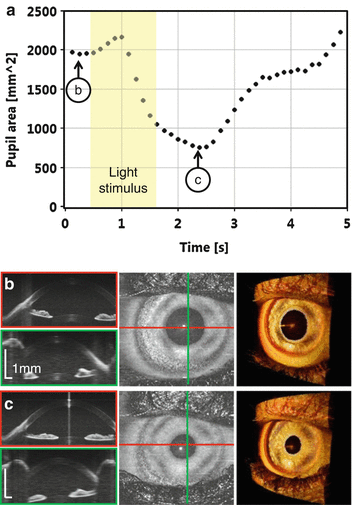
Fig. 10.9
4-D OCT imaging of pupillary reflex. (a) Plot of pupil area versus time measured from 3-D OCT data. Application of light stimulus is indicated in yellow. (b) 3-D rendering, en face OCT, and cross-sectional images extracted from the volumetric OCT data of the eye before light stimulus. (c) 3-D rendering, en face OCT, and cross-sectional images extracted from the volumetric OCT data of the eye at the time of maximum pupil constriction (From Ref. [77])
10.3.5 High-Speed Microscopy and Endoscopy
High imaging speeds which can be achieved with SS-OCT are also important for applications such as microscopy and endoscopy. In conventional microscopy, transverse resolution is determined by the numerical aperture of the objective, which in turn limits the depth of field. OCT has the advantage that it decouples the diffraction-limited axial and transverse parameters of the focused beam. In most OCT applications, high axial resolutions can be achieved with broadband light sources, but the transverse resolution is not sufficient to reveal cellular or subcellular features. Optical coherence microscopy (OCM) combines low coherence detection with confocal microscopy to improve the transverse resolution of OCT images [81, 82]. The utility of OCM to identify pathologies has been demonstrated in ex vivo studies on human breast, thyroid, and renal tissue [81–83]. OCM also has a broad range of applications in research and biological microscopy, ranging from cellular level imaging of the cortex in small animals to in vivo imaging of developmental biology specimens.
OCM has several advantages over traditional confocal microscopy. OCM uses coherence gating to remove out-of-focus light, and compared with confocal microscopy, OCM can image scattering tissues with improved contrast [84, 85]. The imaging depth in confocal microscopy is limited by loss of contrast due to unwanted scattered light and aberrations. The optical sectioning provided by coherence gating significantly improves image quality by removing unwanted scattered light, and OCM enables deeper imaging of biological specimens. OCM was originally developed using time-domain detection, which allows video-rate en face imaging [81]. However, since time-domain OCM (TD-OCM) enables acquisition of only a single coherence-gated depth, both confocal and coherence-gate depths must be carefully matched. This increases the complexity of the system. In addition, variations in path length delay arising from the non-coincident pivot locations of the galvanometer mirrors in scanning microscope systems produce a curved en face image surface which does not match the objective focal plane [86]. Fourier-domain detection enables simultaneous imaging of multiple depths, which reduces the complexity of acquiring en face images and enables the reconstruction of en face images at multiple depths [87, 88]. Post-processing algorithms may be applied to volumetric Fourier-domain OCM data in order to compensate for path length variations across the scan field as well as dispersion mismatch between sample and reference arms [89, 90]. However, since each en face OCM pixel requires the acquisition of an axial scan, ultrahigh imaging speeds are important in order to achieve acceptable en face frame rates. Swept source OCM (SS-OCM) offers ultrahigh speed that is critical for real-time imaging and display to provide diagnostic feedback in clinical settings such as the pathology laboratory or endoscopy suite. SS-OCM systems also require wide wavelength sweep bandwidths to achieve high axial resolution at 1,000 nm and 1,300 nm wavelengths.
High-speed OCT/OCM can be performed using swept source/Fourier-domain detection with a VCSEL light source. VCSEL light sources are well suited for SS-OCM because they can operate at MHz sweep rates and are broadly tunable at 1,000 nm and 1,300 nm wavelengths. The example shows a VCSEL operating at 1,310 nm with a 280 kHz sinusoidal sweep frequency and bidirectional axial scan rate of 560 kHz [31]. A tuning range of 117 nm was achieved, which provided an axial resolution of 13.1 μm in air, corresponding to 8.1 μm in tissue. Fourier-domain detection has the advantage that the dispersion mismatch between the sample and reference arms can be numerically compensated enabling sample arm optics and magnification to be easily changed. As mentioned in Sect.10.2, SS-OCT can be interfaced to a confocal microscope. The microscope had interchangeable objectives (40×, 20× and 10×) for different transverse resolutions (0.86–3.42 μm) and different fields of view (600 × 600 μm2, 1 × 1 mm2 and 2 × 2 mm2). Figure 10.10a shows example ex vivo OCM images of a normal human colon specimen taken with three different magnification objectives demonstrating multi-scale imaging. The specimen can be surveyed with a low magnification 10× objective with large field of view (FOV; 2 × 2 mm2) to show the general architecture of the specimen. With the aid of higher magnification 20× and 40× objectives, details of the colon crypt structures can be visualized. SS-OCM images delineate the mucin secreting goblet cells residing in the crypts and correspond well with features visualized in histology.
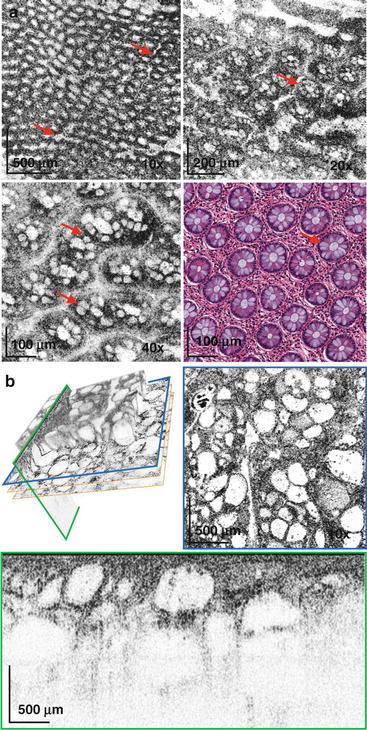
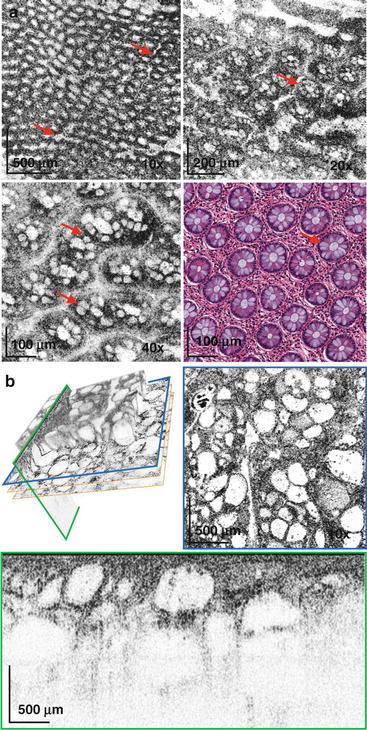
Fig. 10.10
High-speed ex vivo optical coherence microscopy (OCM) using swept source/Fourier-domain detection with a VCSEL light source at 1,310 nm wavelength and 560,000 axial scans per second imaging speed. (a) SS-OCM images of a fresh ex vivo human colon specimen with different magnification objectives. Goblet cells are indicated with arrows. Lower right image shows corresponding histology image of colon tissue for comparison. (b) Volumetric SS-OCM of a fresh ex vivo thyroid specimen. En face OCM images provide high transverse image resolution and uniform signal compared with cross-sectional images (Figure from Ref. [31])
SS-OCM also enables volumetric imaging by simultaneously acquiring signals from multiple en face depths. Figure 10.10b shows a 3-D rendering of a fresh ex vivo human thyroid specimen where different en face images and cross sections can be extracted from the volumetric data, similar to the 3-D ophthalmic images presented previously. As an example, an en face plane from the same volumetric data set was selected 50 μm below the specimen surface. Depth-dependent features of follicular architecture can also be clearly observed in the OCM images. The en face images enable high transverse image resolution, but the ability to extract multiple depths is limited by the depth of field and confocal parameter which trades off against high transverse resolution.
Another approach is to perform image mosaicking to preserve high resolution but also obtain wide field of view. A large specimen area can be imaged at high resolution using a high magnification objective and acquiring multiple partially overlapping volumes with a small field of view which are stitched to generate a large field of view image. Figure 10.11 shows an example of a wide-field SS-OCM image from a fresh ex vivo normal human kidney specimen. The image was generated by combining 30 frames taken with a 40× objective to obtain a 1.8 × 2.1 mm2 total field of view. Glomeruli and convoluted tubules can be observed throughout the imaging field, consistent with the characteristics of normal renal cortex. Detailed examination can be performed by zooming into regions of interest.
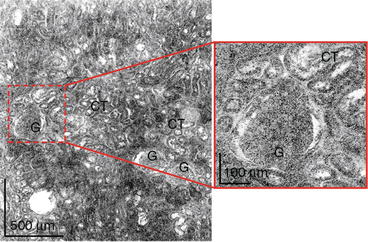
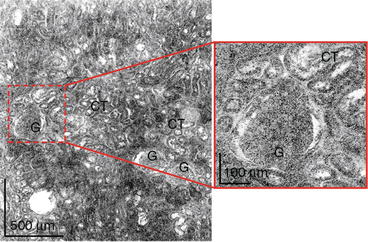
Fig. 10.11
Example mosaic large-field SS-OCM image of a normal human kidney specimen ex vivo. Stitching of 30 individual en face images was performed. CT convoluted tubules, G glomerulus (Figure from Ref. [31])
OCT imaging has also been applied in fiber-optic-based endoscopes [91, 92]. OCT can visualize microstructural features of internal luminal organs to detect pathology associated with disease such as cancer or atherosclerosis. However, in vivo endoscopic OCT imaging is challenging because high-speed optical scanning must be implemented inside a miniaturized imaging probe [93]. Many scanning mechanisms have been realized in catheter-based endoscopic OCT systems, such as proximal rotation of a torque cable and fiber with a distal microprism [91, 94–96], actuating a distal fiber tip using a galvanometer [97], actuating a fiber on a cantilever by piezoelectric transducer [98–100], beam scanning using microelectromechanical systems (MEMS) [101–104], and rotary beam scanning in a tethered capsule [105].
High-speed SS-OCT endoscopy requires high-speed endoscopic scanning devices. This example shows ultrahigh-speed endoscopic SS-OCT imaging using a VCSEL light source at 1,310 nm wavelength with a micromotor imaging catheter [32]. The system imaged at 400 frames per second with 1 MHz axial scan rate, 11 μm axial resolution, 7 μm transverse resolution, and 1.65 mm imaging range in air (corresponding to 8 μm axial resolution, 8 μm transverse resolution, and 1.2 mm imaging range in tissue). The micromotor could operate at 1,200–72,000 rpm (corresponding to 20–1,200 fps). Volumetric data sets were obtained by proximally pulling back the micromotor and optics inside the endoscopic probe sheath in order to obtain a spiral scan pattern. The high frame rate can reduce the total data acquisition time, while distal actuation reduces nonuniform rotation artifacts, improving volumetric data acquisition. Image quality can also be enhanced by averaging consecutive frames to reduce speckle.
High imaging speed enables rapid acquisition of a densely sampled 3-D volumetric data set covering a wide field with minimum motion artifacts in vivo. In the following example, volumetric data in the rabbit colon consisting of 3,000 frames of 2,500 axial scans each was acquired in 7.5 s, covering an area of 7.5 × 7.5 mm2. Figure 10.12a shows volumetric data and representative cross sections. The image quality was improved by averaging three consecutive images perpendicular to the viewing direction. The en face image in Fig. 10.12a shows crypt structures in the colon as well as vessels below the surface (indicated with arrows). The megahertz speed imaging also makes 3-D-OCT acquisition less sensitive to motion, which can be seen in cross sections. Two cross-sectional images are also included in Fig. 10.12a: a cross-sectional image along the longitudinal pullback direction and a cross-sectional image along the rotary direction. The architectural morphology of the colon, such as the epithelium, mucosa, submucosa, and muscular layers can be identified and correspond to representative histology of the rabbit colon.
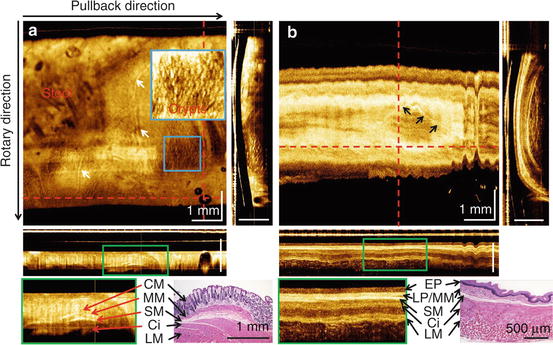
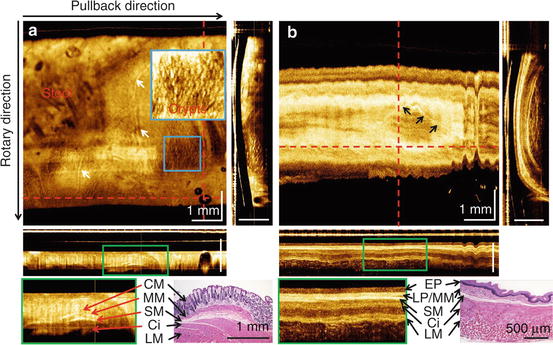
Fig. 10.12
Ultrahigh-speed volumetric SS-OCT endoscopy in the rabbit. The VCSEL light source operated at 1,310 nm wavelength and 1 MHz axial scan rate. (a) SS-OCT images of the rabbit colon. Projection OCT image at 300 um depth. Arrows indicate blood vessels. Cross-sectional images along the rotary and pullback directions. Representative H&E histology of the rabbit colon. (b) SS-OCT image of the rabbit esophagus. Projection OCT image averaged over 15 μm at 190 μm depth. Arrows indicate blood vessels. Cross-sectional image averaged over 12 μm. Longitudinal image averaged over 7.5 μm. Representative histology of the rabbit esophagus. EP epithelium, LP lamina propria, MM muscularis mucosa, SM submucosa, Ci circular muscle, LM longitudinal muscle, CM columnar mucosa (Figure from Ref. [32])
Figure 10.12b shows example 3-D SS-OCT data from the rabbit esophagus. The scanning protocol was similar to the previous example. The cross-sectional OCT images allow visualization of normal esophageal layers including the epithelium, lamina propria/muscularis, submucosa, circular muscle, and longitudinal muscle. The layered structure in the OCT images correlates well with representative histology. The projection view in Fig. 10.12b shows features such as vessels over a large field of view. Although vessels have similar structures to dilated glands in cross-sectional images, they are distinguishable in en face images. Periodic motion due to the cardiac cycle is also visible in the en face view.
10.4 Long-Range Imaging and Optical Metrology
10.4.1 Depth Range in OCT
The performance of Fourier-domain OCT systems is also governed by the axial imaging range (depth range), which is limited by the coherence length of the light source and the highest detectable OCT fringe frequency. The imaging range of the OCT system and light source is often characterized by the −6 dB intensity roll-off depth, i.e., the range at which the interference amplitude signal decreases to one half of its maximum [106].
The imaging range in TD-OCT depends on the mechanical scanning range of the reference arm. However, the imaging range (maximum detectable optical path difference) in SD-OCT systems is limited by the spectral resolution of the spectrometer. Therefore, the spectrometer design (optics, dispersive element, and camera specifications) plays a key role in determining the imaging range in SD-OCT. On the other hand, SS-OCT systems detect interferometric signals in time, and therefore different factors determine the imaging range. The light source coherence length, related to its instantaneous linewidth, is a key parameter which determines the imaging range in SS-OCT. The maximum range which can be measured by an SS-OCT system also depends on other laser and hardware specifications such as sweep rate of the swept light source, the wavelength sweep range, the detector bandwidth, and the sampling rate (bandwidth) of the acquisition (A/D card).
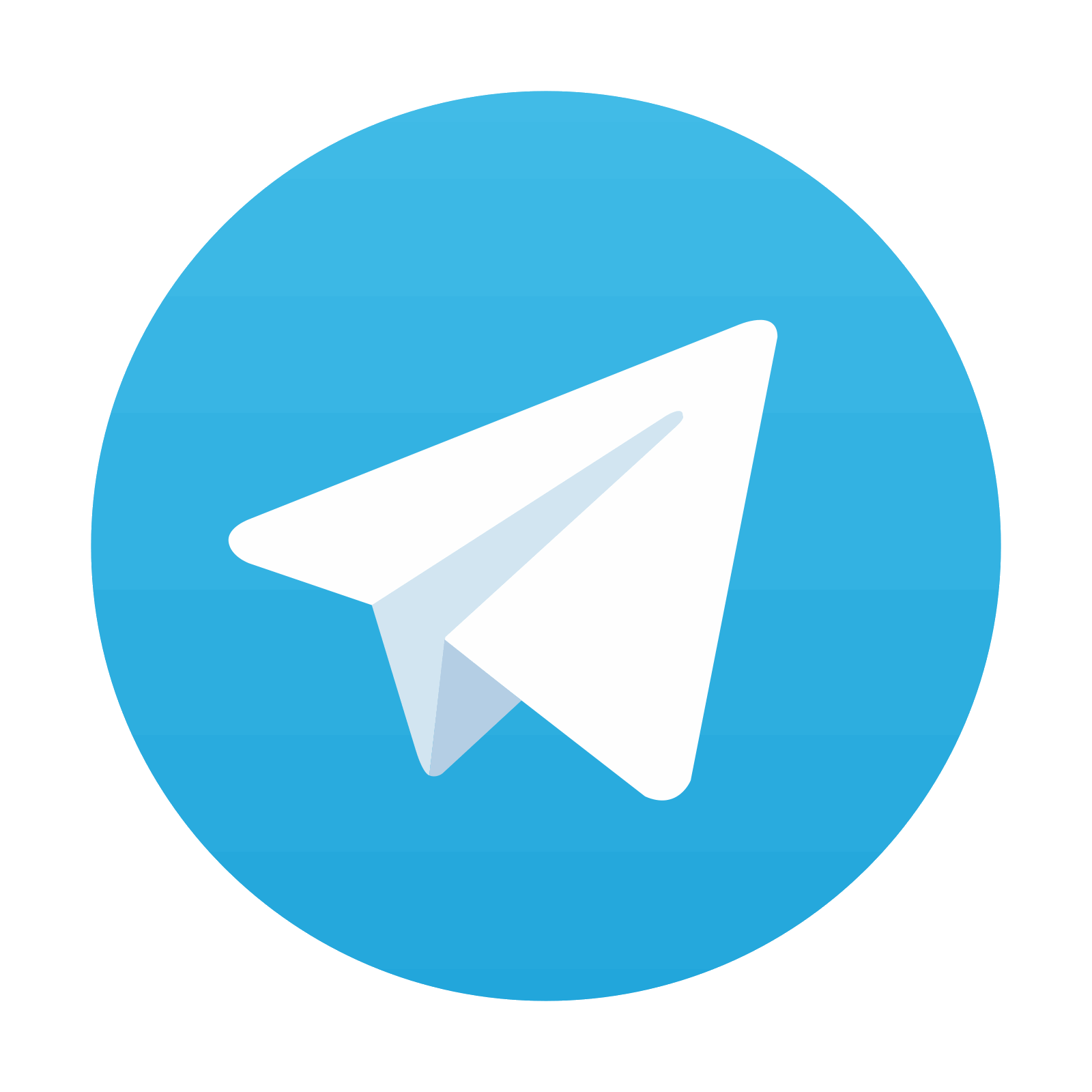
Stay updated, free articles. Join our Telegram channel
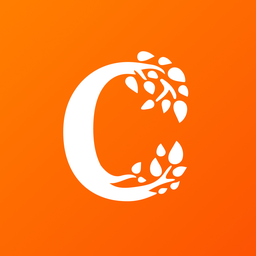
Full access? Get Clinical Tree
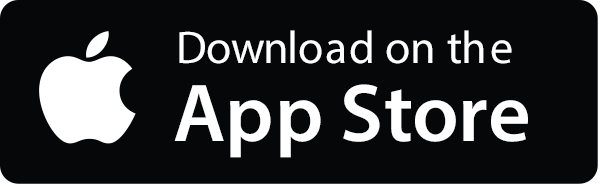
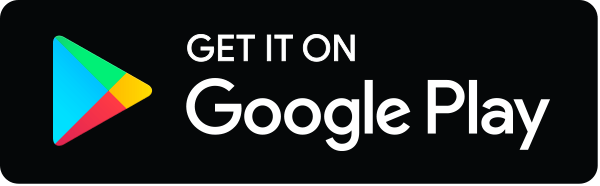