Fig. 7.1
Organotypic cochlear culture. a, b Fixed cochlear wholemounts viewed at low (a, scale bar = 100 µm) and high (b, scale bar = 20 µm) magnification. Spiral ganglion (SG) and organ of Corti (OC) are indicated in a. Structural elements shown in b are indicated by adjacent text under the panel. c A z-projection of a confocal image stack of a fixed organotypic cochlear culture at low magnification (scale bar = 50 µm) labeled with anti-HMW neurofilament (NF200, red) to show SGNs and their axons and with anti-myosin 7A (blue) to show hair cells. d A z-projection of a confocal image stack of a fixed organotypic cochlear culture at high magnification (scale bar = 20 µm) labeled, as in c, to show axons and hair cells and with anti-PSD95 (green) to show synapses. Structural elements shown in d are indicated by adjacent text above and below the panel. e A z-projection of a confocal image stack of a fixed organotypic cochlear culture at high magnification (scale bar = 10 µm) labeled to show hair cells (anti-myosin 6/7A mix, blue), presynaptic ribbons (anti-CtBP2, red), and postsynaptic densities (anti-PSD95, green)
Cell and tissue cultures require incubation in medium containing all essential nutrients at physiological temperature and with physiological CO2 concentration. For organotypic preparations there are additional considerations. The organ must attach itself to the substrate for support. The thickness of the cultured tissue impedes exchange of O2, CO2, nutrients, and metabolites between the culture medium and the tissue. The methods first described by Fell for successful maintenance of organ cultures were used by other investigators with little modification for several decades. To facilitate gas, nutrient, and metabolite exchange, the organ is placed on a porous substrate to which it can attach. In the earliest studies, substrates used included cellulose sponge, sterile fabric, paper, cellophane, or gelfoam. Fell’s original protocol for culture of organs, including the otocyst, was to place the embryonic organ on a small clot formed of chick embryo extract and plasma at the bottom of a small glass centrifuge tube. The organ was transferred daily to a fresh medium and could be fixed and stained for microscopic observation.
Lawrence and Merchant (1953) followed up on Fell’s study some 25 years later with observations of chick embryo otocyst development and differentiation in vitro. Their method was similar to that of Fell but they added a porous substrate—cellulose sponge—to which the otocyst attached to prevent the tissue from rounding up and so obtained morphogenesis more closely resembling that in vivo. They described development of all vestibular and auditory sensory epithelia. Lawrence and Merchant also reported culture of a single mammalian (rat) otic vesicle using a protocol similar to that used for the chick and observed formation of a cochlear duct and organ of Corti. However, Lawrence and Merchant did not observe full organ and cell differentiation in their chick or rat preparations and did not culture their preparations longer than 8 days.
Starting in 1956, Friedmann produced a series of publications investigating development of the chick embryo inner ear in organotypic culture. Friedmann’s initial publication (Friedmann, 1956) used methods similar Fell’s but the culture was on a clot in a shallow watch glass rather than in a centrifuge tube. He also included more of the surrounding mesenchyme when dissecting out the otocyst. The result was an even greater degree of differentiation of the auditory and vestibular sensory epithelia than that observed by Fell.
Up to this time, observations had focused on differentiation of the sensory structures but not innervation. Indeed, Lawrence and Merchant (1953) described efforts to remove the statoacoustic ganglion (SAG) “to have the vesicle as clean as possible.” (In early embryonic development, the presumptive auditory and vestibular neurons form a continuous mass of cells, the statoacoustic ganglion, also termed the cochleovestibular ganglion [CVG] that later subdivides into the spiral ganglion and Scarpa’s/vestibular ganglion.) The first ultrastructural study of inner ear development in vitro was that of Friedmann (1959) and this paper also provided the first observation of innervation in vitro, describing nonmyelinated axons extending past supporting cells into the sensory epithelium and forming contacts on hair cells. In this study Friedmann cultured the otocysts on small convex strips of voile, a sheer fabric, which supported the otocyst while allowing gas exchange.
A further technical refinement was that of Reinecke et al. (1960), who cultured chick embryo otocysts supported on cellophane strips between two glass coverslips in a Rose perfusion chamber—which allowed light microscopic observation of development of the live otocyst over periods of time up to 3–4 months after which the otocysts were fixed and stained. Reinecke et al. described differentiation of the sensory epithelium and, in cultures in which the neurons were included, growth of axons into the sensory epithelium.
Subsequent studies by Friedmann and Bird (1967), Friedmann (1968, 1969) and by Orr (1965) described in more detail innervation and synaptogenesis in organotypic cultures of chick embryo otocysts. Orr’s (1965) paper was the first to focus specifically on the acoustic ganglion. In this, she used a culture system similar to that of Reinecke et al. (1960) in that the otocysts were placed directly on a coverslip in a chamber. The cultures were maintained for up to several months, observed and photographed daily by light microscopy, and ultimately fixed and stained. Orr further systematically tested a variety of culture media with respect to cell survival, neuronal differentiation, and myelination. She described neuronal differentiation and subsequent degeneration of a small number of neurons, rapid growth of axons into the sensory epithelium, and gradual myelination of a subset of the axons by Schwann cells.
Friedmann’s studies pioneered the use of electron microscopy to study otocyst development in vitro. He described cell differentiation on the ultrastructural level including that of hair cells and neurons. Friedmann showed in vitro differentiation of characteristically morphologically distinct types of hair cells in auditory and vestibular sensory epithelia and “type I” and “type II” hair cells in vestibular epithelium. He showed presynaptic structures including vesicles and “bars” (ribbons) adjacent to postsynaptic terminals. With regard to neurons, he showed differentiation into morphologically distinct types resembling type I (myelinated), type II, and “intermediate” neurons adjacent to the sensory epithelia and formation of “cup-shaped” or “chalice-shaped” calyx and “bud-shaped” bouton postsynaptic terminals on, respectively type I and type II vestibular hair cells. These events closely resemble in vivo development.
Subsequent technical developments are the use of “tissue culture” dishes—dishes made of specially treated plastic to promote cell adhesion—in many cases coated with extracellular matrix proteins such as laminin, and improved formulations of culture media and non-serum supplements that have permitted some reduction in the serum concentration—although supplementation of the media with serum levels 10 % or greater has remained a common feature of organotypic cochlear cultures. This is in spite of variations among laboratories in substrates and culture media adopted for particular experimental requirements.
As methods of observation of fixed preparations have developed, fluorescence microscopy has been added to bright-field microscopy with conventional stains and to electron microscopy. Fluorescence microscopy allows selective visualization of specific molecules and cell types by immunofluorescence (see Fig. 7.1d, e for examples) or expression of appropriate fluorescent proteins. As noted previously, the earliest studies used bright-field and phase-contrast microscopy (Fig. 7.1a, b) to observe cell movements in live preparations. More recently, differential interference contrast is used and, as described in Sect. 7.2.6, expression of fluorescent proteins in live cells.
7.2.2 Innervation of the Mammalian Organ of Corti in Vitro
Early efforts at long-term organotypic culture of the mammalian otocyst were unsuccessful in that significant degeneration occurred in the tissue (Lawrence & Merchant, 1953), so studies prior to and throughout the 1960s were restricted to chick embryos. A comprehensive and systematic effort by Van De Water and Ruben (1971) resulted in identification of culture conditions for mouse embryonic otocysts that maintained healthy tissue and permitted morphogenesis and cell differentiation of sensorineural elements of the inner ear, including afferent synapses on hair cells (Van de Water et al., 1973; Van De Water & Heywood, 1976). Use of mammalian cultures, including human spiral ganglion cultures (Rask-Andersen et al., 2005), allows studies more directly relevant to human health as well as exploitation of mouse genetic technology—first in studies of genetically deaf mice and, later, of transgenic mice.
These early studies highlighted requirements for proper support and substrate and for serum-supplemented medium for organotypic culture of the cochlea from avian and mammalian sources. With appropriate culture conditions, key aspects of in vivo sensorineural development, including neuronal and hair cell differentiation and synaptogenesis, proceed in vitro. Moreover, the early studies showed that conditions can be identified for microscopic observation of live tissue over time as well as for fixation and labeling of the tissue at any time. These laid the foundation for use of in vitro approaches to investigate important questions regarding innervation of the organ of Corti and the related trophic and inductive interactions among SGNs and cells of the organ of Corti.
7.2.3 Trophic Support of Spiral Ganglion Neurons by the Organ of Corti
A hypothesis that peripheral auditory or vestibular neurons depend on inner ear sensory epithelium for trophic support is consistent with the observation that postnatal destruction of hair cells with an aminoglycoside antibiotic results in a gradual degeneration and death of the spiral ganglion neurons in vivo (Spoendlin, 1975). Asking whether this is also the case during development and determining the mechanism, required experiments with organotypic cultures. It should be noted that at the time of these observations, neurotrophic factors other than the nerve growth factor (NGF)—which does not play a role in the cochlea (Green et al., 2012)—had not yet been characterized, although by the early 1980s there was increasing evidence that neurotrophic factors other than NGF existed and could exert trophic or tropic influence on neurons (Barde et al., 1983).
Ard et al. (1985) tested the trophic effect of their peripheral and central targets on the survival of chick embryo cochleovestibular ganglion neurons in vitro. Explants consisting only of SAG showed relatively poor neuronal survival even in serum-supplemented and elevated potassium medium. In contrast, organotypic explants consisting of SAG with otocyst or SAG with medulla or SAG with both otocyst and medulla showed greatly increased neuronal survival. The otocyst alone provided better trophic support than did the medulla alone, but the presence of both otocyst and medulla did not increase survival significantly over otocyst alone. These experiments indicated that some neurotrophic factor(s) are available to SAG neurons from both pre- and postsynaptic sources but with additional factor(s) available only from the presynaptic sensory epithelium. Experiments in which the ganglion and otocyst were first separated from each other and then co-cultured suggested that the trophic factor is either not diffusible or, if so, operates at close range.
Experiments with mouse embryo otocysts and ganglia cultured for two weeks on hydroxyethylmethacrylate hydrogels (Zhou & Van de Water, 1987) showed results comparable to the experiments with chick embryos. In explants consisting of ganglion with attached sensory epithelium, ganglion with attached hindbrain, or ganglion with attached sensory epithelium and hindbrain, neuronal survival was evident as was axon growth into the epithelium and the hindbrain tissue. Neuronal survival was approximately equal in these three types of explants; there was no indication of better trophic support by the sensory epithelium than by the hindbrain as had been observed with chick embryo explants (Ard et al., 1985). In contrast, neuronal survival in explants consisting only of the ganglion was less than 20 % of that in ganglion with a pre- or postsynaptic target. Thus, both pre- and postsynaptic targets provide neurotrophic support to the cochleovestibular neurons.
Although these experiments could not identify the neurotrophic factors involved—a work still in progress—they did show that such factors exist and provided valuable information on their range and relative efficacy. Soon after the neurotrophic factors brain-derived neurotrohic factor (BDNF) and neurotrophin 3(NT-3) were discovered and shown to be expressed in inner ear sensory epithelium (Pirvola et al., 1992; Ylikoski et al., 1993), experiments using chick embryo SAG explants showed that BDNF and NT-3 were trophic to SAG neurons and promoted neurite outgrowth (Avila et al., 1993). Subsequent experiments, described in Sect. 7.3.3, used dissociated spiral ganglion cultures to identify neurotrophic factors—for example, NT-3, BDNF, GDNF—sufficient to support SGN survival (Malgrange et al., 1996; Hegarty et al., 1997). Studies showing that these factors promote SGN survival in vivo are summarized by Fritzsch et al. in Chap. 3. Experiments testing whether some of these factors are necessary for SGN survival, at least in development, have been performed using knockout mice in which the genes encoding BDNF or NT-3 or their receptors have been genetically deleted; these experiments are also discussed by Fritzsch et al. in Chap. 3.
Barclay et al. (2011) used cultures from P1–P7 mice maintained on polylysine-coated tissue culture plastic; explant cultures consisting of an isolated portion of the spiral ganglion or organotypic cultures consisting of a portion of the spiral ganglion attached to the corresponding portion of the organ of Corti, with innervation remaining intact. Addition of BDNF promoted SGN survival. In the absence of added BDNF, survival of SGNs in isolated ganglia was poor; in organotypic cultures, the presence of organ of Corti appeared to result in rescue of type II, but not of type I, SGNs. However, Wang and Green (2011) did not detect SGN death over a longer culture time in P5 rat organotypic cochlear cultures similarly consisting of organ of Corti and associated ganglion. Poor SGN survival in some cultures may therefore be an artifact of the culture conditions, which can be demanding for organotypic cultures. The Wang and Green study included higher serum concentrations and culture on laminin, a more physiological substrate, which may be necessary for SGN survival in organotypic explants.
7.2.4 Trophic/Inductive Effects of the SGNs on the OC
Experimental manipulations of organotypic culture and co-culture systems were used to establish that there is a trophic effect of inner ear sensory epithelium on SAG neurons. Similar methods can be used to ask the reverse: Is innervation necessary for survival or differentiation of hair cells? Some early studies using chick embryo otocysts suggested that development of the sensory epithelium and differentiation of hair cells is improved by the presence in the explant of SAG tissue (Friedmann, 1968; Orr, 1968). This may be related to deficiencies in the culture conditions or to differences between avian and mammalian cultures. Van de Water (1976) compared organotypic cultures of embryonic mouse otocysts that either contained or did not contain SAG and found no difference between them in morphology of the organ of Corti, of vestibular sensory epithelia, and of the hair cells. These were cultured on tissue culture plastic with a high (20 %) serum concentration. This latter study implies that initial differentiation of inner ear sensory structures during embryonic development is independent of innervation but again implies that cell survival and differentiation in organ cultures is sensitive to culture conditions, especially substrate and serum concentration.
7.2.5 Guidance Factors for Spiral Ganglion Neurite Growth in the Cochlea
Organotypic cultures have been used to determine whether inner ear sensory epithelium can provide neurite guidance for neurons. Evidence that otocyst sensory epithelium can provide a chemotropic signal that attracts SAG neurites from a distance was shown in experiments with organotypic cultures (Van De Water & Ruben, 1983, 1984). In these experiments, otocysts from 11-, 12.5-, or 14-day mouse embryos, either including or lacking the ganglion, were cultured on filters in tissue culture wells adjacent to an isolated SAG. All otocysts that included a ganglion had innervation of the sensory epithelium showing that a local guidance signal was produced by the sensory epithelium. Otocysts lacking a ganglion taken from 11- and 12.5-day mouse embryos were innervated, showing that they could release a signal that attracted neurites from the separate SAG. However, otocysts from 14-day mouse embryos were unable to attract neurites from a separate SAG, implying that the chemotropic signal is available only during the time when innervation normally occurs but is not produced later. Electron microscopic observations of the vestibular sensory epithelium confirmed formation of synapses between hair cells and those neurites attracted to the otocyst (Anniko & Van de Water, 1986).
Bianchi and Cohan (1991) used chick embryo otocysts co-cultured with SAG neurons and confirmed the presence of an otocyst-derived factor that promoted neurite growth from SAG neurons. Moreover, production of the factor by the otocyst declined as development progressed from embryonic day 4 to day 13, consistent with the observation of a developmental decline in production of a chemotropic factor by mouse otocysts from embryonic day 11 to day 14 (Van de Water & Ruben, 1984). Bianchi and Cohan further showed (Bianchi & Cohan, 1993) that the factor was not one of the neurotrophic factors identified by that time—that is, not a neurotrophin or CNTF—and elicited responses only in SAG neurons and not in other peripheral neurons. More recently, this otocyst-derived chemotropic factor has been identified by Bank et al. (2012) as the inflammatory cytokine macrophage migration inhibitory factor (MIF), which promotes neurite growth from chick and from mouse SAG neurons. The MIF receptor, CD74, is present on embryonic SAG neurons and on adult mouse SGNs (Bank et al., 2012).
Another important example of the use of in vitro techniques to investigate guidance of SGN axons is investigation of the role of ephrin–Eph signaling (Cramer, 2005; Coate & Kelley, 2013). Both ephrin to Eph (“forward”) signaling and Eph to ephrin (“reverse”) signaling are typically repulsive to axons, contributing to axon guidance by preventing axons from growing toward inappropriate targets. Expression of Ephs and ephrins has been investigated during embryonic development in chick (Siddiqui & Cramer, 2005) and mammalian (van Heumen et al., 2000) cochleae. Although the patterns of expression differ among chick, mouse, and guinea pig (van Heumen et al., 2000), the patterns suggest Eph to ephrin “reverse” signaling as a repulsive cue for cochlear neuron axon guidance in the chick and the mouse.
This possibility was tested directly by experimentally manipulating ephrin signaling in vitro. Chick SAG neurons express ephrin-B1 and activating these receptors on cultured SAG neurons with soluble EphB–Fc fusion proteins inhibited neurite growth (Bianchi & Gray, 2002). Mouse SGNs express ephrin-B2 and ephrin-B3, both of which interact with EphA4, which is expressed in fibroblasts of the spiral ligament and presumptive osseous spiral lamina (van Heumen et al., 2000). Brors et al. (2003) showed that EphA4 does indeed provide a repulsive cue to SGN axons, using mouse spiral ganglion explants cultured in tissue culture wells coated with stripes of different molecules: SGN neurites were repelled by EphA4-containing stripes. Coate et al. (2012) further showed, using co-cultures of SGNs with otic mesenchyme, that reducing EphA4 expression (by knockdown of the transcription factor Pou3f4) resulted in a failure of the otic mesenchyme to promote fasciculation of SGN neurites. Addition of soluble EphA4–Fc fusion proteins to the culture restored fasciculation. These experiments suggest that EphA4 in otic mesenchyme, particularly in the presumptive osseous spiral lamina, repels SGN peripheral axons, forcing them to fasciculate. For reasons not yet explained, fasciculation of these axons is necessary for normal synaptogenesis on inner hair cells (IHCs; Coate et al., 2012).
Brugeaud et al. (2014) showed that a known chemorepulsive cue, Repulsive Guidance Molecule A (RGMa), is expressed in the mouse inner ear throughout development as well as postnatally. Addition of a blocking antibody for RGMa to postnatal organ of Corti–SGN co-cultures increased the number of synapses formed between hair cells and SGN neurites and accelerated the maturation of SGN neurite morphology.
7.2.6 Direct Observation of Spiral Ganglion Neurite Growth in the Cochlea
As summarized in Sect. 7.2.1, direct observation of innervation of sensory epithelium in embryonic otocysts in vitro was accomplished more than half a century ago (Reinecke et al., 1960; Orr, 1965). However, the behavior of individual axons and growth cones cannot be easily observed by conventional light microscopy. The axons are bundled into fascicles in which individual axons are difficult to distinguish. Also the borders of all of the other cell types—for example, epithelial, mesenchymal, glial—in an organotypic explant obscure the thin axons in unlabeled live tissue. An elegant use of mouse genetics, combined with an innovative modification of organotypic culture, was used by Appler et al. (2013) to observe the behavior of live axons in the developing cochlea. They expressed a fluorescent protein, eGFP or tdTomato, selectively in neurons using a Cre recombinase driven by a neuron-specific promoter. Expression of the fluorescent protein only in neurons allows clear visualization of live axons in organotypic cultures by confocal microscopy without interference from other cell types. Appler et al. dissected embryonic cochleae from the transgenic mice and cultured them in serum-containing medium on filter paper in a glass-bottom (coverslip thickness) culture dish. This allowed continuous confocal imaging over many hours through a small hole in the paper over which the cochlea was placed.
An example of the use of these methods was the demonstration of aberrant axon trajectories in SGNs lacking the transcription factor Gata3. Normally, SGN axons grow from the ganglion toward the sensory epithelium with little deviation from a straight radial path. Time-lapse observation of live axons shows that for SGNs lacking Gata3 the axon trajectories clearly deviate from a radial direction and may exhibit occasional turns rather than being straight (Appler et al., 2013). The conclusion is that this transcription factor regulates expression of genes required in SGNs for sensing axon guidance cues normally used to attract SGNs to the sensory epithelium and form synapses. Further studies (Yu et al., 2013b) have shown that the transcription factor MafB acts downstream of Gata3, in particular in regulating gene expression required for normal synapse formation on IHCs. It is not yet known whether these are related to chemotropic cues explored in the studies summarized in Sect. 7.2.5, for example, macrophage MIF (Bank et al., 2012).
A further innovation is to use a “leaky” promoter (Druckenbrod & Goodrich, 2014) that results in expression of the fluorescent protein in only a small random subset of SGNs. This “sparse” labeling facilitates time-lapse observations of individual axons, even in fascicles, using culture and confocal microscopy methods like those used by Appler et al. (2013). Preliminary observations using such methods show that SGN axons initially grow rapidly through the mesenchyme toward the organ of Corti and fasciculate with the earliest emerging axons. On reaching the organ of Corti, the axons slow their growth and exhibit exploratory behavior with transient branching and contacts with inner and outer hair cells (OHCs). These dynamic changes in growth cone behavior indicate different guidance cues in different regions of the cochlea along the axon’s path. Axon growth can be observed in live cultured SGNs from species other than mouse by expression of fluorescent proteins from genes introduced into the SGNs by transfection or viral transduction (Atkinson et al., 2011).
7.2.7 Regional Specification of SGN Physiological Phenotype
SGNs exhibit heterogeneity in several physiological and cellular characteristics, which have been reviewed (Davis & Liu, 2011; Green et al., 2012) and discussed by Davis and Crozier in Chap. 4. These include significant differences along the tonotopic (base to apex) axis in the cochlea. As summarized in these reviews, apical and basal SGNs can differ in aspects of membrane electrophysiology, expression of voltage-gated ion channels including K+ channels and Ca2+ channels, expression of α-amino-3-hydroxy-5-methyl-4-isoxazolepropionic acid (AMPA)-type glutamate receptor subunit types and expression of some presynaptic proteins. To test the hypothesis that SGN regional identity along the tonotopic axis is induced by factors in the corresponding region of the organ of Corti, organotypic explants of apical or basal organ of Corti were co-cultured with explants of apical or basal spiral ganglion in immediate proximity (Flores-Otero et al., 2007). Because these physiological properties that differ among SGNs are relevant to their function in hearing, cultures in these studies were made from neonatal, as opposed to embryonic, cochleae. The tissues were cultured in tissue culture wells in serum-containing medium.
By these means, Flores-Otero et al. demonstrated that apical organ of Corti induced an apical phenotype in SGNs—higher synaptophysin immunoreactivity—in a NT-3–dependent manner; conversely, basal organ of Corti induced a basal phenotype in SGNs—that is, higher GluA2/3 immunoreactivity—in a BDNF-dependent manner. Addition of NT-3 to cultured spiral ganglion neurons induced an apical phenotype in SGNs while addition of BDNF induced a basal phenotype with respect to membrane electrophysiological properties, expression of voltage-gated channels and AMPA-type glutamate receptors, and expression of presynaptic proteins (Adamson et al., 2002a; Zhou et al., 2005; Flores-Otero et al., 2007). This is consistent with the higher level of NT-3 expression in the apical organ of Corti relative to the basal in postnatal mice (Sugawara et al., 2007).
7.2.8 Regeneration: Studies of Postnatal Cultures
The studies summarized in Sects. 7.2.2–7.2.7 pertain to aspects of the development of cochlear innervation and have largely been confined to embryonic tissue. More recently, studies have been initiated using organotypic cultures from postnatal mice or rats to investigate trauma that affects cochlear innervation and regeneration of innervation after such trauma. Organotypic culture of postnatal cochleae poses an additional challenge relative to embryonic cochleae: more care is required in the dissection because of the tougher surrounding bone and connective tissue. This is not much of a problem in the first postnatal week as the bone has not yet ossified. Starting in the second postnatal week, dissection of a completely intact and undistorted explant in which all cells remain viable in long-term culture becomes increasingly difficult with increasing age. For this reason, current studies of postnatal organotypic cochlear explant cultures or cochlear tissue co-cultures have generally used mouse or rat pups in the first postnatal week.
Organotypic culture of postnatal cochlea was initially described by Sobkowicz et al. (1975), and the methods she developed have been reviewed in detail (Sobkowicz et al., 1993). These investigators cultured intact cochlear explants from neonatal mice consisting of the organ of Corti with a portion of attached spiral ganglion for up to 27 days, although not all SGNs survived that long and intact morphology of the explant was generally not retained for more than 2 weeks. Nevertheless, they observed a normal pattern of innervation by surviving SGNs, with both radial and spiral fiber bundles. Innervation of the apex was completed, indicating that neurite growth continued in vitro.
In the protocol used by Sobkowicz et al. (1975), the culture medium contained a high proportion of serum (initially 40 %). The sensory elements and spiral ganglia were removed intact but cut into pieces prior to plating to obtain segments that do not coil in the culture dish and that adhere well to the substrate. The explants were cultured on glass coverslips coated with collagen (predominantly type I collagen), a substrate commonly used in cell culture. More recent studies have used similar methods with glass coverslips coated with extracellular matrix components, although laminin has proved more appropriate than type I collagen (Parker et al., 2010; Wang & Green, 2011; Tong et al., 2013) and is now generally used. In this regard, it should be noted that cochlear basal laminae are rich in laminin and collagen IV (Santi & Johnson, 2013).
Culture on a glass coverslip allows the specimen to be labeled and then observed on a microscope with an inverted stage without being moved or disturbed, thus preserving intact morphology. Sobkowicz et al. (1975) used bright-field microscopy with compatible staining, relying primarily on silver staining optimal for labeling neurons and axons. Culture on glass coverslips is also optimal for imaging by current methods of confocal microscopy. Sobkowicz et al. (1975), Rose et al. 1977) described fibers emerging from the SGN somata, projecting peripherally to the organ of Corti in radial fascicles, and becoming myelinated. These studies reported that most of the neurons were unipolar, retaining only the radially projecting processes, indicating that the centrally directed axons degenerated after the auditory nerve was severed when the cochlea was dissected and placed in culture. However, subsequent studies have shown that with proper culture conditions central axons are preserved and become myelinated with time in culture (Sobkowicz et al., 1993).
After methods for culturing postnatal cochleae were developed, studies followed on the consequences of trauma and possibility of regeneration (Rose et al., 1977; Sobkowicz & Slapnick, 1992). These described the consequences to SGN peripheral axons of mechanical injury to the explant that occurred in the culture. After displacement of the hair cells that did not result in severing the axons, the axons increased in length, presumably to relieve the tension due to the mechanical stretch, but retained the original connections. Greater displacements that caused the axons to become “disconnected”—typically breaking near the base of the hair cell— resulted in many axons growing freely, at a high rate, and in a manner apparently unrestricted by the sensory epithelium. These axons tended to extend radially past the sensory epithelium and then take apparently random paths (Rose et al., 1977). In a study using electron microscopy that focused primarily on rapid responses—within 4 h—following mechanical disruption of axons in neonatal mouse cochleae after 3 days in culture, Sobkowicz and Slapnick (1992) observed that growth cones of regenerating axons that were close to hair cells did form synapses on the hair cells. “Orphan” ribbons could be observed in the hair cells, apparently due to failure of many axons to regenerate synapses although the number of regenerated synapses was not counted in the study.
Although such an injury does not reflect typical inner ear trauma or pathology, the results are informative about the capacity of postnatal SGNs to grow neurites or even reestablish connections with hair cells. The results imply that, after trauma sufficient to sever their axons or disconnect the axons from hair cells, the damaged ends of axons of postnatal SGNs are able to acquire growth cones and elongate. However, the observation that many, if not most, axons grow past the sensory epithelium and do not track to hair cells, implies that postnatal sensory epithelium does not provide cues sufficient to guide the axons back to the hair cells or to regenerate synapses on the hair cells. Such a conclusion is consistent with the in vitro studies of chemotropic signaling, discussed in Sect. 7.2.5, showing that the sensory epithelium provides a chemoattractant signal to SAG neurons during embryonic development when innervation is occurring but that this signal is not present in late embryonic sensory epithelium. Nevertheless, synapse regeneration is evidently possible if the growing axon does encounter a hair cell.
7.2.9 Excitotoxic Trauma to Cochlear Synapses and Relevance to Noise Trauma
Disconnection of SGNs from hair cells in vivo can be the result of what, unfortunately, may be a very common type of trauma: noise trauma. Pujol et al. (1985), Puel et al. (1995) showed that intracochlear perfusion with the non–N-methyl-d-aspartate (NMDA) glutamate receptor agonist kainic acid (KA) caused swelling and degeneration of synapses on IHCs or “synaptopathy,” a type of excitotoxic trauma. Spoendlin (1971) and Pujol, Puel, and colleagues (Puel et al., 1998) noted similar swelling and loss of synapses on IHCs as a consequence of noise trauma. These observations suggested that noise damage to synapses is excitotoxic, that is, loud sound causes excessive release of the excitatory neurotransmitter glutamate from hair cells, which causes excitotoxic trauma to the synapses. In support of this hypothesis, the damage to synapses occurring after noise is prevented by kynurenic acid, a nonselective ionotropic glutamate receptor antagonist (Puel et al., 1998) while inhibition of glutamate reuptake, which increases exposure of the synapses to glutamate, exacerbates damage (Hakuba et al., 2000). Kujawa and Liberman (2006, 2009) quantified the consequences of such “moderate” noise levels and showed a reduction in the number of synapses on IHCs (OHCs do not appear to be affected.) Comparable results were found in guinea pigs (Lin et al., 2011). This could explain the observation that “moderate” noise levels, which do not destroy hair cells nor cause a permanent threshold shift, may nevertheless cause permanent hearing impairment and damage to the cochlea.
Pujol, Puel, and colleagues reported synapse regeneration in vivo after excitotoxic trauma (Puel et al., 1995) or after noise (Puel et al., 1998). This is consistent with the previous in vitro experiments (Sobkowicz & Slapnick, 1992) that suggested there was some capability of synapse regeneration after damage in vitro if the regenerating axon was in close proximity to the hair cell, as it would be after excitotoxic damage to the postsynaptic terminal. All of these observations of synapse regeneration were made by electron microscopy, making it difficult to quantify the extent of regeneration. Confocal microscopy with immunofluorescent detection of synaptic proteins allows detection of all synapses on many hair cells in a single image stack, facilitating quantitation. Using confocal microscopy, Kujawa, Liberman, and colleagues did not detect any increase in the number of synapses on IHCs in vivo starting 3 days after noise exposure (Kujawa & Liberman, 2009).
To determine whether synapse regeneration can occur and what neurotrophic factors might promote regeneration, Wang and Green (2011) used an in vitro system that facilitates experimental analysis of excitotoxic trauma to cochlear synapses (Fig. 7.1). The methods used were similar to those originally used by Sobkowicz and colleagues to produce intact organotypic cochlear explant cultures containing a portion of the organ of Corti and the attached portion of spiral ganglion (Sobkowicz et al., 1975, 1993). Because synapses had to be counted, care was taken to ensure that the explant was intact with all cells present and viable and all synapses maintained. Neonatal rat or mouse pups were used (Wang & Green, 2013). Explants were cultured on cover glasses coated with polyornithine and laminin, in medium containing 20 % serum to improve viability. The explants were placed with the basilar membrane side down in the well, as this assists in getting them to flatten and adhere to the substrate, so preserving the morphology of the explant. Most of the medium was removed for 1–2 h, leaving only a thin film (~50 µL) on the explant, a step that has been shown to promote attachment (Parker et al., 2010). Firm attachment to the cover glass allowed labeling and confocal microscope imaging without moving the specimen, which would risk physically distorting it. Excitotoxic trauma is accomplished by exposure to the glutamate agonist KA. This recapitulates key features of excitotoxic or noise-induced synaptopathy in vivo, in particular loss of most synapses on IHCs but with those on OHCs unaffected (Pujol et al., 1985; Kujawa & Liberman, 2009). Excitotoxic trauma in vitro, like that in vivo (Puel et al., 1994), is mediated by non–NMDA-type glutamate receptors: exposure to KA in vitro results in almost complete loss of synapses, whereas inclusion of NMDA with KA has no additional effect (Wang & Green, 2011).
In this in vitro system, a limited degree of reinnervation and synapse regeneration can be detected after excitotoxic trauma, restoring approximately 12 % of synapses by 72 h post-trauma (Wang & Green, 2011). “Orphan” ribbons were found in the hair cells, presumably as a result of the persistence of ribbons in the absence of complete reinnervation, an observation made previously by Sobkowicz and Slapnick (1992). Addition of neurotrophins—NT-3 or BDNF—to the culture increases the number of synapses up to 43 % of the pre-trauma number. The endogenous NT-3 produced by the hair cells appears to be crucial for synapse regeneration on IHCs after excitotoxic trauma (Wang & Green, 2011, 2013).
In summary, use of this organotypic culture system has shown that reinnervation and synapse regeneration is possible, at least in neonatal rodents, after excitotoxic trauma has destroyed innervation of IHCs. It has also revealed dependence of reinnervation on neurotrophic factors, particularly NT-3. There is also potential for this system to be used to investigate the mechanism of excitotoxicity. Experiments using this or similar organotypic cochlear explant culture systems may be useful for prevention or recovery from noise damage.
7.2.10 Reinnervation After Replacement of Lost Hair Cells or SGNs
Prevention of excitotoxic/noise-induced synaptopathy or reinnervation after synaptopathy are situations in which in vitro studies of cochlear synapse regeneration may result in therapeutic benefits in the near future. Looking further into the future, one can envision at least two potential situations in which synapse formation on hair cells in adults will also be a necessary therapeutic goal. One is innervation of new hair cells derived from gene or stem cell therapy to replace lost hair cells (Kesser & Lalwani, 2009). The second is innervation of existing hair cells by SGNs regenerated from gene or stem cell therapy described by Nayagam and Edge in Chap. 9.
Data from the studies summarized in Sect. 7.2.8 indicate that synapse formation is possible in the postnatal rodent cochlea and can be investigated in vitro using organotypic cultures. Further support for this possibility is derived from more recent studies using co-cultured explants. Flores-Otero et al. (2007) placed micro-isolates of inner and OHCs adjacent to SGN clusters to demonstrate synaptogenesis in the presence of NT-3 and BDNF. Martinez-Monedero et al. (2006) used β-bungarotoxin to eliminate SGNs from an organotypic cochlear explant culture and then co-cultured the remaining organ of Corti with spiral ganglion neurons to determine whether these could innervate the hair cells. This experimental design is formally similar to that originally described by Van De Water and Ruben (1983, 1984), summarized in Sect. 7.2.5, that tested chemotropic attraction of SAG neurites to otocyst sensory epithelium but, in the Martinez-Monedero et al. (2006) study, instead done using cultures from postnatal mice.
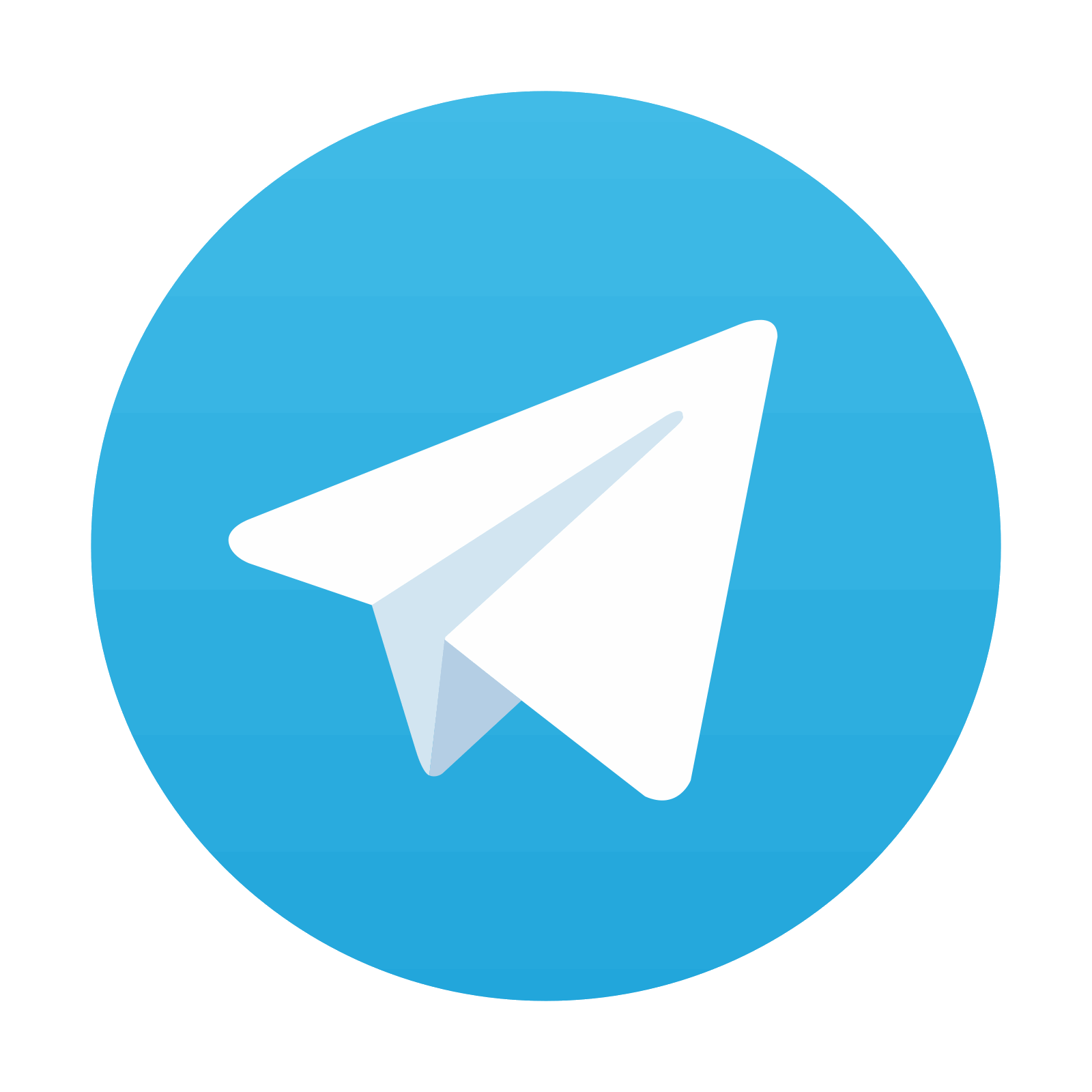
Stay updated, free articles. Join our Telegram channel
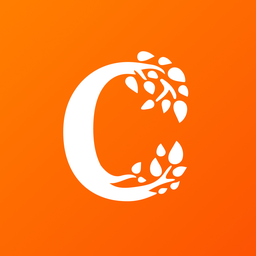
Full access? Get Clinical Tree
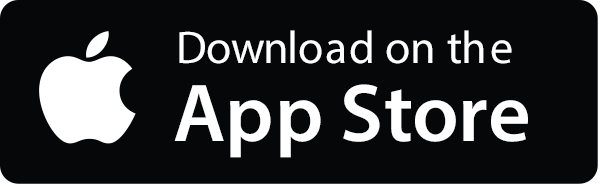
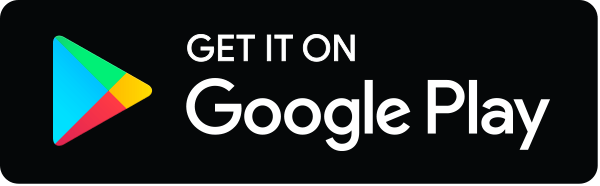