Fig. 5.1
Summary graphic describing the stages of progenitor cell (blue) to retinal ganglion cell (green) transition, and the transcription factors and extrinsic, environmental signals known to be associated with each stage. Math5 and Notch are known to regulate cell cycle exit but are not sufficient on their own to specify RGC fate (see text and Fig. 5.2)
How does a developing RGC integrate into its environment? RGCs are among the first neurons to arise from a common pool of multipotent retinal progenitors in the retinal neuroblast during embryonic development. After exiting the cell cycle, RGCs migrate across the retina to the ganglion cell layer (GCL). In the GCL, RGCs extend axons towards the optic nerve head and form the optic nerve which ultimately connects the eye to the brain. RGCs proceed to form synaptic connections with presynaptic amacrine and bipolar interneurons [13–15]. It remains unclear what signals regulate neural integration, or to what degree each step—cell fate specification, survival, migration, neurite growth, and synaptic integration—depends on the preceding one (see Fig. 5.1).
What signals regulate retinal progenitor competence to specify RGC fate determination ? Through seminal “birth-dating” experiments, two well-defined but overlapping RPC competence states for generating specific types of neurons, including RGCs, were identified [16]. “Early” embryonic retinal progenitors differentiate into early-born retinal neurons beginning with RGCs and amacrine, cone photoreceptor and horizontal cells [17, 18]. This is followed by an overlapping shift in cell competence to commit to late-born retinal cells, including rod photoreceptors, bipolar, and Müller glia [10]. How these competence changes occur is not well understood but evidence suggests that these differential competence states are strongly influenced by intrinsic mechanisms. Time-lapse experiments suggest that RPC competence is intrinsically programmed and linked directly to temporal context, albeit with some stochastic component [19]. Heterochronic transplantation experiments in both chick and rodents, in which progenitors from different stages of development transplanted to an environment of a different age/context (either earlier or later) support this premise [20]. For example, early chick progenitors, which normally generate RGCs in vivo, retain their competence for RGCs irrespective of the age of the surrounding environment. Experiments with cultured rat progenitors showed that early progenitors that typically generate RGCs, amacrine cells, and cone photoreceptors do not lose this competence when cultured in different environments known to secrete inductive signals for other neural fates [20]. This suggests that RPC competence to produce distinct types of cells differs depending on the stages of development, independent of environmental context. Similarly controlled spatiotemporal waves of changing cell competence have been observed in many areas of the CNS, including cortex and hippocampus. While early RPCs retain their early-born neuron competence, late RPCs can be influenced by environmental signals. Late progenitors cultured in the presence of early retinal conditioned media were coaxed into the RGC fate, demonstrating that cell competence changes can change in specific directions [21]. Taken together, cell competence for the RGC fate is intrinsic to the early retinal progenitor and decreases during development in a discrete temporal window in order to establish the appropriate cell numbers.
Besides cell competence, cell cycle and cell division mechanisms in retinal progenitors also change over time during development and greatly influence cell number and fate. Cell-cycle duration doubles throughout retinal neurogenesis [22]. Furthermore, the type of cell division shifts over time in retinal progenitors. During early retinal development there is considerable generation of mitotic progenitors, as large numbers of cells divide symmetrically, each giving rise to two progenitors. As development progresses the generation of new progenitors decreases, concomitant with the increased generation of post-mitotic neurons. Cell polarity and the orientation of the cell division plane correlate with proliferation and cell determination in the developing cortex and in the retina [23]. Thus, asymmetric segregation of cell-fate determinants during cell division may play an important part in generating cell diversity in vertebrate retina. For example, the asymmetric segregation of the protein numb, occurring only in a precise cell division plane, plays a role in cell fate determination in the rat retina [24]. Thus, cell competence for specific neural subtypes, including RGCs, is dynamically regulated by cell autonomous, environmental, and cell polarity signals which work in concert to define the temporal window in which specific cells are born. As reviewed below, disruption of any of these signals has adverse effects on retinal development.
Transcription Factors Regulate RGC Fate
Through numerous gain and loss of function experiments both in vitro and in vivo, transcription factors and secreted factors have been shown to regulate the specification and differentiation of RGCs, but precise instructive signals remain unknown (Figs. 5.1 and 5.2). On the cell-autonomous side, transcription factors are master regulator proteins which regulate expression of downstream gene targets. Transcription factors, particularly the basic helix loop helix (bHLH) and homeodomain families, have been shown to control the differentiation and patterning of many of the diverse cell types in the CNS, and specific gene regulatory pathways are required to complete and progress through a series of developmental stages. In a hierarchical manner, transcription factors regulating early developmental processes are important early, while transcription factors regulating terminal differentiation and later maturation processes are important later. Some of the main transcriptional regulators within this hierarchy, including Pax6, Six3, Rx, Chx10, Notch, Ath5, and the Brn3 family of transcription factors, have been identified and examined extensively, but the genes these transcription factors target and how these signals work in together remain unknown.
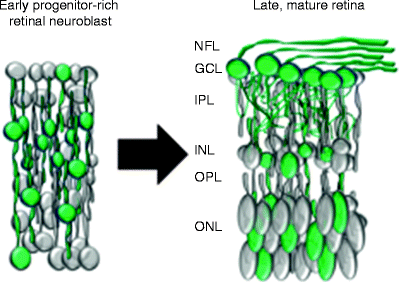
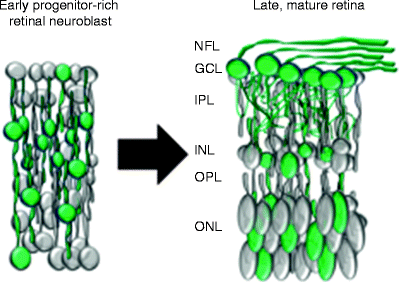
Fig. 5.2
Math5-positive progenitor cells (green, left) differentiate into RGCs and many other retinal neurons found in the mature retina (green, right), suggesting that Math5 is not sufficient on its own to specify RGC fate. (NFL nerve fiber layer, GCL ganglion cell layer, IPL inner plexiform layer, INL inner nuclear layer, OPL outer plexiform layer, ONL outer nuclear layer)
Pax6
Loss-of-function studies with knockout mice have placed certain homeobox-containing transcription factors at the top of gene regulatory networks controlling retinal development. These genes include Pax6, Rx, Chx10, and Six3, which are all expressed in RPCs in the retinal neuroblast. These homeobox genes are expressed in all RPCs in the beginning of retinogenesis and are required for the specification of RGCs as well as other retinal cell types. Pax6 is required for eye field specification during the early stages of eye development [25] and also for generating cell types in the developing retina [26]. In seminal gain of function experiments in drosophila, later shown in vertebrates, Pax6 was sufficient to trigger the cascade of signals required for eye formation [25]. Conversely, elimination of Pax6 function by a conditional knockout in the developing retina led to a loss in the specification of all retinal cell types excluding amacrine cells. Pax6 functions, at least in part, to promote the expression of the bHLH proneural genes in retinal neuroblasts, as loss of Pax6 results in the decreased expression of genes encoding the proneural bHLH factors Ath5, Ath3, and neurogenin [26]. NeuroD expression is unaffected by the loss of Pax6, consistent with evidence from knockout mice establishing that NeuroD is crucial for amacrine cell differentiation [27]. Taken together, these data demonstrate that multiple sets of transcription factors regulate RPC proliferation and differentiation during retinal neurogenesis, which begins with RGCs, and corresponds with the upregulation of Ath5 expression.
Ath5
Through loss-of-function experiments in mouse, the proneural bHLH gene Ath5 (also called Xath5 in Xenopus, Cath5 in chick and Math5 in mouse) was demonstrated to be necessary but not sufficient for RGC fate (Fig. 5.3). During development, Ath5 is expressed almost exclusively in the retina. In the mouse retina, Math5 expression begins directly before the birth of the first RGC and its expression decreases in daughter RGCs soon after RGC precursors exit the cell cycle [28]. The importance of Ath5 in the RGC lineage was recognized through both gain- and loss-of-function studies. Overexpression of Cath5 in chick retinas [29] and Xath5 [28, 30] in Xenopus retinas stimulates RGC production at the cost of generation of other retinal cell types. Null mutations in Ath5 in mice [31, 32] and zebrafish [33] lead to almost complete absence of RGCs. Although Ath5 is essential for RGC formation, evidence suggests that it is probably upstream of the instructive signals for RGC specification, as lineage-tracing experiments show that Ath5-expressing RPCs give rise to multiple cell types [31]. Ath5 likely acts as a proneural gene to promote the establishment of a field of progenitor cells that are competent to turn into RGCs but not to specify the RGC lineage. Ath5 overexpression, which increases the number of RGCs, may do so by generating a larger pool of RGC progenitors competent to then differentiate into RGCs. Taken together, evidence suggests that Ath5 is necessary but not sufficient to specify RGC fate, and that Ath5 may not specify a particular cell type at all, but rather is more involved in multiple steps of retinal neurogenesis, including the differentiation of RGCs and other cell types in the retina.
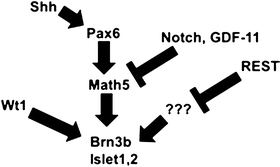
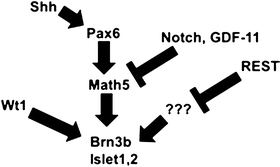
Fig. 5.3
General overview of known factors regulating retinal ganglion cell fate specification. See text for details
Notch
During development, pro-neurogenic signals compete with opposing signals to coordinate the generation, distribution and patterning of newly born neurons. Opposing RGC fate, Notch has been shown to be a key regulator of cell fate in the CNS and plays an important role as a negative regulator of RGC production [34, 35]. The Notch signaling pathway is activated by the binding of ligands such as Delta, typically through neighboring cell–cell interaction. Activation of this pathway leads to the proteolytic cleavage of Notch and the release of a Notch intracellular domain (NICD). NICD translocates to the nucleus and binds to the highly conserved DNA-binding transcription factor CSL to activate target genes, including the Hairy-Enhancer of Split (HES) family of bHLH genes [36, 37]. Evidence from Drosophila studies demonstrates that the Notch pathway negatively regulates neurogenesis in the developing eye through lateral inhibition resulting in the repression of the proneural bHLH gene atonal [38]. In the vertebrate retina, the Notch pathway is similarly positioned at the top of the regulatory hierarchy in RGC generation and inhibits in RGC production through lateral inhibition. Pax6 and the Notch pathway compete with each other in regulating downstream genes required for the generation of the RGC lineage. Pax6 is required for the activation of Ath5 [39], which is required for the RGC lineage. Conversely, Notch signaling inhibits ath5 expression through its downstream target transcription factors, Hes1 and Hes5. It is unknown whether ath5 is a direct transcriptional target of Pax6 and/or Notch–CSL, or whether other regulating signals are required in these pathways. Taken together, these data suggest that Pax6– and Notch-dependent mechanisms, in concert with other signals, fine-tune the proper levels of ath5 expression in a subset of progenitor cells that become competent for RGC specification.
Brn3
Downstream of these pathways, Brn3 proteins (also called XBrn3 in Xenopus) Brn3a, b, and c are class IV POU domain transcription factors and one of the initial and most specific markers for RGC differentiation during development [40]. Around 80 % of RGC precursors express Brn3b immediately after cell cycle exit, and 24 h later the closely related Brn3a and Brn3c genes are expressed in ~80 and ~20 % of developing RGCs, respectively. These latter two subsets of RGCs significantly overlap the subset of Brn3b expressing RGCs [41–44]. These transcription factors have been shown to control dendritic stratification, axonal structure and target selection during the terminal differentiation stage and their expression patterning may control the development of unique subtypes of RGCs (see Fig. 5.1) [45].
Of the Brn3 proteins , Brn3b has been best studied, and gain- and loss-of-function experiments indicate that Brn3b is directly downstream of ath5 in the regulatory hierarchy for RGC differentiation. Although Brn3b can promote the expression of certain RGC markers when overexpressed [46], there is strong evidence that demonstrates that Brn3b itself is not a required cell fate specification gene for RGCs. In Brn3b-null retinas, the number of RGCs born initially resembles that observed in wild-type retinas [40]. Therefore, this suggests that there are probably unknown gene regulatory pathways that function in parallel to Math5 and upstream of Brn3b.
In math5 -null retinas, Brn3b expression is greatly reduced [31, 32], consistent with the absence of RGCs. However, it remains unknown whether Math5 directly regulates Brn3b expression. In the retina, the spatial and temporal expression patterns of Math5 and Brn3b are largely non-overlapping. Furthermore, Math5 is expressed in proliferating progenitor cells and Brn3b is expressed in postmitotic RGC precursors and mature RGCs [31, 40]. If Math5 directly upregulates Brn3b expression early on, other mechanisms must be responsible for maintaining high levels of Brn3b expression after Math5 expression declines during retinal development. In Brn3b-null retinas, lacZ knocked into the Brn3b locus mirrors the normal expression pattern of Brn3b [40]. These findings suggest that maintenance of Brn3b expression is not likely controlled by autoregulation. It is possible that genes required for RGC specification lie in between and/or parallel to Ath5 and Brn3b in the regulatory hierarchy and that these unknown specification genes either collaborate with or function independently of Ath5 to regulate expression of Brn3b. Interestingly, knocking out both Math5 and the transcriptional repressor RE-1 silencing transcription factor (REST) in mouse retina leads to the generation of ectopic Brn3b/Islet1 double-positive RGCs [47]. This further demonstrates that Brn3b expression does not depend entirely on Math5 expression. Currently it is unknown if REST is repressing uncharacterized cell fate specifying transcription factors, but evidence from experiments in other parts of the CNS suggests this hypothesis [47].
Wt1
The Wilms’ tumor gene (Wt1), which encodes a zinc-finger transcription factor, was found to function directly upstream of Brn3b in the retina [48]. Wt1-null mice have a major loss of RGCs in the retina which, similar to Brn3b-null mice, initially generates RGCs that are later lost by apoptosis. Wt1 expression does not overlap with Ath5 expression and it is not clear whether Ath5 regulates Wt1. Wt1-dependent activation of Brn3b could be part of an Ath5-independent signaling cascade regulating RGC differentiation. Although Brn3 family members and Wt1 transcription factors do not play role in specifying RGC fate from RPCs, it is likely that they signal important downstream targets for the full RGC phenotype, which may need to be upregulated in stem cell-derived RGCs if transplantation is to be considered (discussed further below). Thus there remains a gap in our understanding of RGC fate regulation between upstream transcription factors like Ath5 and Pax6 that are necessary but not sufficient, and downstream transcription factors required for RGC maintenance or survival.
Secreted Molecules Regulate RGC Fate in Concert with Transcription Factors
During development, secreted molecules from the local environment work in concert with transcription factors to induce commitment to subsequent steps in differentiation [49]. Secreted factors such as fibroblast growth factors (FGFs), sonic hedgehog (Shh), and transforming growth factor beta (TGF-β) superfamily molecules have been shown to regulate cell number and the timing of neural differentiation by regulating transcription factor expression [50–52].
Basic Fibroblast Growth Factor
The trophic factor and mitogen basic fibroblast growth factor (bFGF) has been shown to potentiate RGC fate determination in mammalian retinal progenitors [53, 54]. In an RPE transdifferentiation assay, bFGF elicits the expression of RGC marker RA4, although the extent of differentiation may be very limited [29, 55], because those cells did not express many other RGC markers. Expression of these markers was detected in bFGF-primed RPE cultures infected with RCAS–Ath5 or RCAS–NSCL1 [29], suggesting that the bHLH hierarchy may integrate input from bFGF to promote RGC differentiation and development. Consistent with previous findings that FGF promotes the retinal neurogenic pathway [53, 56], blocking FGF receptor activation in chick interferes with the progressive wave RGC differentiation from the central retina towards the periphery [57].
Sonic Hedgehog
Sonic hedgehog is another extrinsic factor shown to regulate proliferation and RGC generation and differentiation [58–61]. Recent studies have established a mitogenic role for Shh signaling in CNS progenitor cells. For example, cerebellar granule cell precursors depend on Shh secreted by Purkinje cells to proliferate in vitro and in vivo [50, 62, 63]. In early retinogenesis, Shh derived from the first-born RGCs promotes propagation of the neurogenic wave front [59] but suppresses RGC genesis as these neurons accumulate, as discovered in zebrafish [59]. Shh secreted by RGCs appears to also negatively affect RGC generation through a different feedback system [64]. Thus Shh regulates the precise number of RGCs generated during development through at least two mechanisms. It remains unclear as to whether the morphogenic property of Shh observed in other areas of CNS development plays a role in regulating these contrasting modes of function.
Shh signals also appear to influence the growth and trajectory of RGC axons [65, 66]. In zebrafish, reduction of Hh activities affects differentiation of late cell types including Müller glia, bipolar cells, GABAergic amacrine cells, and photoreceptors [61, 67]. Furthermore, laminar organization of the retina is disrupted in Shh mutants [67, 68].
Recently, Shh has also been implicated in adult neural stem cell proliferation [69]. Mice with a single functional allele of the Shh receptor patched have an increased percentage of proliferating cells in their retinas throughout the first postnatal week. In addition, the mice have a population of dividing cells at the retinal margin reminiscent of the ciliary marginal zone (CMZ) of lower vertebrates [70]. This suggests that Shh signaling is important for controlling retinal progenitor proliferation and may regulate adult neurogenesis in the mammalian eye. Taken together, Hh signaling, perhaps due to its morphogenic properties, is fundamentally important to many facets of RGC differentiation, including postembryonic ocular growth, but how these mechanisms function together remains unknown.
Growth Differentiation Factor 11
In the retina, feedback regulation of neural cell number, mediated by secreted factors, has been shown to alter the fates of multipotent progenitor by controlling the timing of transcription factor expression. For example, the secreted TGF-β molecule growth differentiation factor 11 (GDF11) negatively regulates the number of neuron generated by controlling the period during which retinal progenitor cells are competent to produce particular progeny. The GDF11 KO mouse has aberrantly persistent Math5 expression throughout postnatal development resulting in the generation of excessive numbers of RGCs. [52]. Conversely, exposing retinal explants to GDF 11 results in the decrease in Math5 expression resulting in retinas with less RGCs. It is currently unknown which cell type(s) secrete GDF11 as well as whether other GDFs play roles in retinal development. Manipulation of these signaling pathways could provide insight into improving the methods for the generation of donor RGCs for transplantation.
Cell Choices for Transplantation
What types of stem or progenitor cells can be used for RGC therapies in glaucoma or other optic neuropathies? The most comprehensively studied donor cell candidate for cell-based therapies in the retina has been embryonic stem cells (ESCs), which proliferate, self-renew and differentiate into all cell types. In culture, ESCs retain all of these features. ESCs have been differentiated in culture into most retinal cell types including RGCs [71–73].
However, transplantation studies of undifferentiated ESCs have demonstrated that these cells fail to receive the proper instructive cues for correct cell fate specification. Transplanting an uncommitted stem cell will rely heavily on the host tissue to provide differentiation cues to the grafted cells and so far for RGCs, this has yielded only minimal re-integration with no evidence for any functional restoration. Further limitations to using ESC s directly come from observations in some studies in which the cells formed tumors due to uncontrolled proliferation following transplantation [74]. Other challenges may include immune rejection, teratogenic properties, and ethical concerns over the cell source. Thus, control of proliferation and differentiation of these cells is critical before ESCs are safely and ethically used as a cell source for therapeutic transplants.
Neurons and neural stem cells (NSCs) induced from ESCs and transplanted into the injured eye may show more promise for retinal integration [75–78]. Similarly, ESCs differentiated into retinal stem cells (RSCs) as well as various neuronal phenotypes by exposure to pro-neural differentiation factors in vitro prior to transplantation were investigated in a retinal transplantation model [79, 80]. RSCs subretinally transplanted into young mice survived, migrated, integrated, and differentiated into retinal cell types, particularly rod photoreceptors. However, in adults, transplanted RSCs preferentially expressed RGC or glial markers. These findings provide evidence that RSC differentiation following transplantation hinges on the pre-transplantation condition of both the donor RSCs and the host retina.
NSCs from other areas of the CNS, particularly forebrain, have also been investigated as potential donor cell sources to the retina. Upon transplantation to the retina, forebrain-derived NSCs survive, express some retinal cell specific markers, and exhibit retinal cell-like morphologies. Overall, the rate of integration was low and many of the cells were localized in aberrant retinal layers. As with RSCs, the extent of differentiation and integration depend heavily on the age and type of injury to the host retina [81]. Hippocampal-derived NSCs incorporate into injured retina and differentiate into both microtubule-associated protein 2 (map2) positive and GFAP-positive cells, suggesting differentiation into both major types of cell lineages. Although neurons and glial markers were present, no retinal-specific subtype markers were observed, demonstrating that the local retinal environment, either normal or injured, is not sufficient to coax hippocampal NSCs towards retinal cell types.
Bone marrow stem cells (BMSCs), which are far more easily attained than ESCs, also have restricted potential and offer potential therapeutic promise [82–85]. Even though BMSCs are not linked to neural lineages, they have been coaxed to produce neuron-like cells which express some retinal markers [86]. Following transplantation into the subretinal space, BMSCs generate progeny that express limited retinal markers [87]. However, there is substantially more promise in using BMSCs to produce blood vessels which may be useful to replace vasculature lost in various retinal diseases, or to support the survival of degenerating neurons. For example, following transplantation of BMSCs into the retina, profound revascularization of retina was observed, which resulted in enhanced survival of retinal neurons in models of retinal injury [88–90]. Evidence from these studies further suggests that the improved circulation in these ischemic animal models provide an enhanced conduit for trophic factor delivery which can enhance neuroprotection.
The adult human eye itself contains progenitor cells, which may be influenced towards RGC fate [91, 92]. In lower vertebrates, such as teleosts, the ciliary marginal zone (CMZ) contains stem cells which persist following development and generate new neurons in the continually growing adult teleost retina [93]. Similarly, cells in the ciliary body and a subpopulation of Müller cells in the human retina have been shown to exhibit stem cell-like properties [94–96]. In injury models in lower vertebrates, Müller cells generate new RGCs that then regenerate their axons down the optic nerve [97, 98]. In mammals, neither ciliary body nor Müller cells proliferate in response to retinal injury. However, they display proliferative and multipotent capacity in vitro [99], and in adult mice, optic nerve injury by transection or crush increases cell proliferation and the expression of RPC markers in both the ciliary body and in Müller glial cells and astrocytes in the retina [100, 101]. Thus, stem cells residing in adult tissue, when expanded in vitro and differentiated into the appropriate cells, may enable autologous transplantation-based therapy by using the patient’s own eye as the donor cell source.
Another source of stem cells that could be patient-specific is induced pluripotent stem cells (iPSCs). Through reprogramming adult somatic cells back to an embryonic stem cell-like state, iPSCs were first generated and described in 2006 [102–104]. These cells have advantages over ESCs as a potential donor source including obviating ethical debate over ESC use. In similar differentiation paradigms used for ESCs, iPSCs demonstrated the capacity to differentiate into RPCs, and all the retinal cell type progeny including RGCs [105–107]. However, extensive research still needs to be done to eliminate some of the safety issues associated with these cells [108]. For example, because iPSC s can be developed from a patient’s own somatic cells, initially it was expected that treatment with iPSCs would circumvent immunogenic responses; however, recent evidence has challenged this notion [109]. Furthermore, the specific methods for reprogramming adult cells to obtain iPSCs may pose significant risks that could limit their clinical use. For example, if viruses are used to reprogram cells in iPSCs, the expression of oncogenes may simultaneously be activated. Recently, generation of iPS cells without introducing genes but rather with repeated treatments of specific proteins was shown to be an effective method for reprogramming somatic cells [110]. Whether iPS cells can differentiate into functional replacement cells for use in the treatment of glaucoma or other progressive diseases specific to the visual system remains unknown and certainly an important area for investigation.
Thus a number of stem cell sources may be available for therapeutic development for glaucoma. They may have different advantages and disadvantages including accessibility, reproducibility, patient-specificity, and importantly potential for toxicity. As important will be figuring out what capacity each has to help in RGC degenerative disease, and for that, stem cells may have two important uses: replacing RGCs, which will require differentiation and integration into the retina and visual pathway, or protecting RGCs from death, neuroprotection (Fig. 5.4). Next we address progress being made on these two fronts.
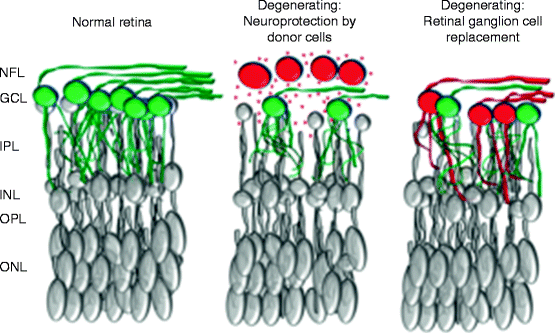
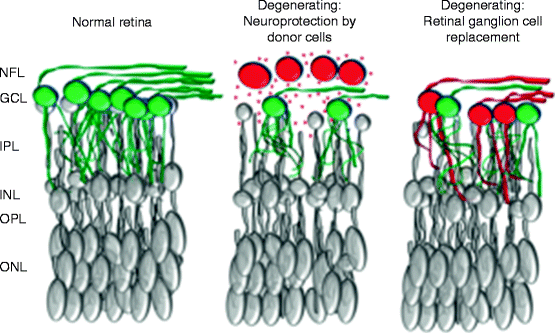
Fig. 5.4
Retinal ganglion cells RGCs (green cells) degenerate and die in glaucoma and other optic neuropathies (middle and right), leaving fewer RGCs than in the normal retina (left). Stem cells or cell therapies (red) for glaucoma or other retinal ganglion cell degenerations could be used for neuroprotection of residual through trophic support (red triangles) or for cell replacement therapy (right). (NFL nerve fiber layer, GCL ganglion cell layer, IPL inner plexiform layer, INL inner nuclear layer, OPL outer plexiform layer, ONL outer nuclear layer)
Cell Transplantation for RGC Replacement
The majority the research on retinal cell transplantation has concentrated on pathologies involving photoreceptor degeneration [76–78]. Lessons from recent studies on photoreceptor replacement approaches suggest that cells further along in differentiation may be more promising than stem and progenitor cells in neuronal cell replacement therapy [111–114]. For example, MacLaren et al. transplanted dissociated retinal cells, including progenitors and post-mitotic retinal cells, from various donor ages subretinally in mouse and found that that the post-mitotic rod precursors rather than multipotent progenitors were capable of synaptically reintegrating in the photoreceptor layer. They found that donor cells from ages which marked the peak birthdates of rods had the most profound highly structured morphological and synaptic integration. The transplantation of Nrl+ immature rod cells was capable of improving visually evoked potentials in genetic models of mouse photoreceptor degeneration. Thus, we are only beginning to understand the importance of the spatiotemporal state of a cell which can be exploited to generate donor cells with the greatest potential for neuroprotection and/or integration.
Compared to phot oreceptors, there has been less progress made in integrating donor cells for RGC replacement. Transplantation of retinal progenitors from various donor ages do not appear to generate newly born and integrated RGCs in vivo [115–119]. Unlike photoreceptors, RGCs extend lengthy axons to specific targets in the brain in addition to making complex dendritic connections with their synaptic partners in the retina. Additionally, in order to be clinically applicable, enhancing graft integration by altering the host retina must be accomplished without disturbing regular retinal function. To add to the complexity, there are many different types of RGCs, each with highly specialized properties which coordinate complex visual functions and likely draw on synaptic plasticity for wiring during development. Successful replacement of RGCs may require differentiation into specific cell subtypes with highly specialized properties, the establishment of numerous synaptic inputs, and the extension of an extremely long axon to precise brain targets in a manner that preserves the retinotopic map. As such, various groups are currently trying to understand how to coax cells in vitro to produce cells that are further along in differentiation, on the potential premise they may be a more transplantable cell source [114]. Presently, it has not been addressed whether purified RGCs, obtained acutely from the retina or derived from stem cells in vitro, can integrate into the normal or injured adult retina, or at what age or stage during post-mitotic development maximizes donor RGC integration following intraocular transplantation.
< div class='tao-gold-member'>
Only gold members can continue reading. Log In or Register a > to continue
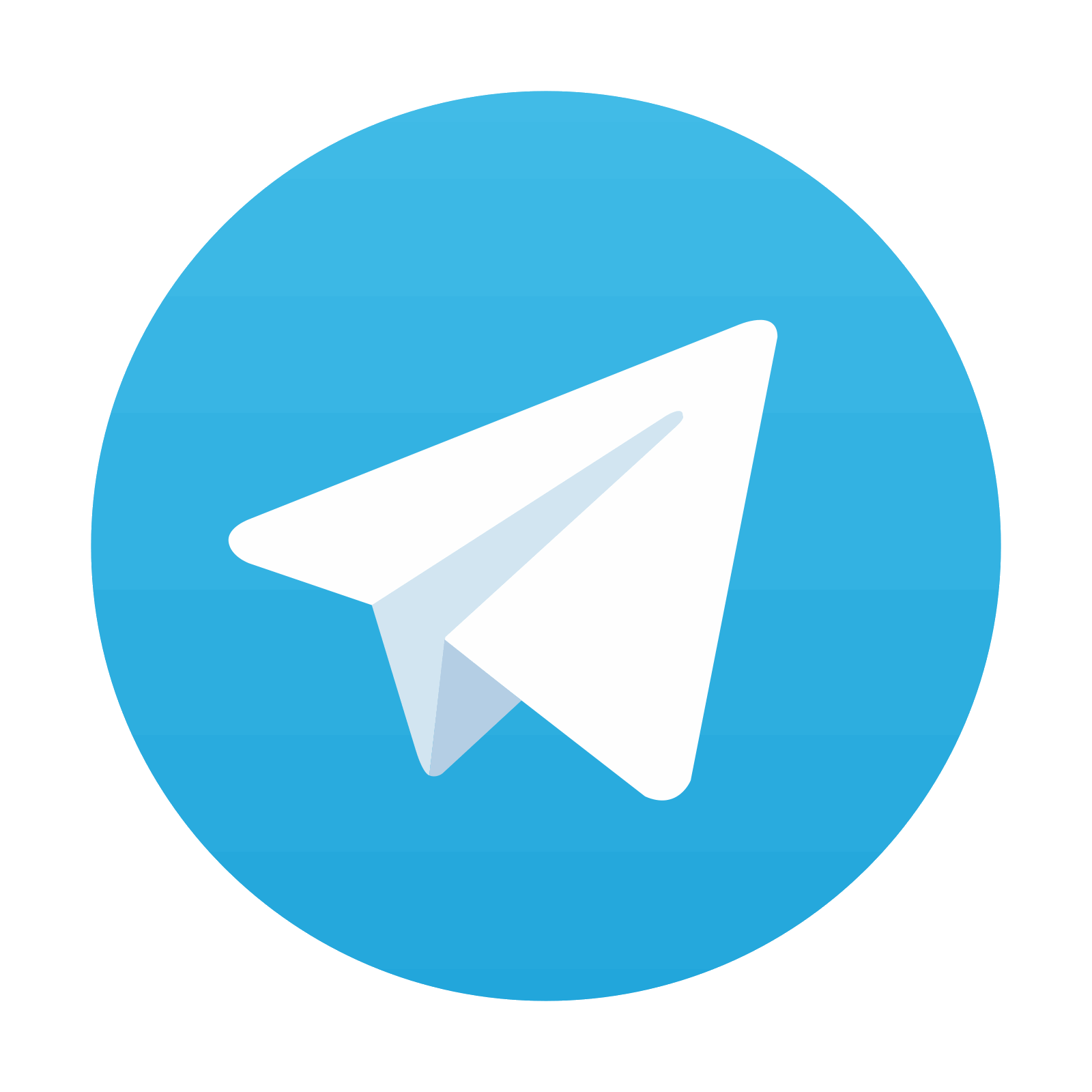
Stay updated, free articles. Join our Telegram channel
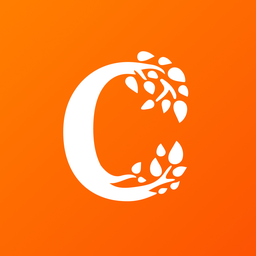
Full access? Get Clinical Tree
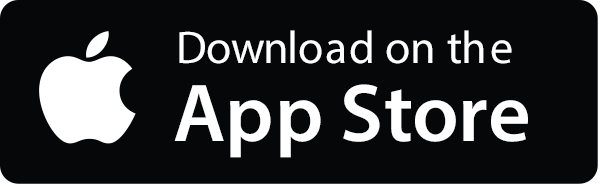
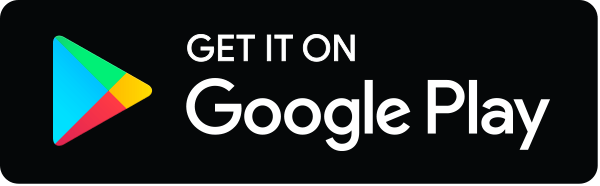