Fig. 4.1
Diagrammatic summary of a three-step procedure to differentiate human ES cells into lens progenitor-like cells and lentoid bodies. (a) Diagram of three steps: noggin treatment (days 0–6), BMP4/BMP7/FGF2 treatment (days 7–18), and differentiation in the presence of FGF2 (essential factor) and Wnt3a (modulatory factor) (days 22–35). Formation of putative cell populations including the neuroectoderm, pre-placodal region (PPR) and neural crest (NC) cells is indicated. (b) Sequential activation of PAX6, αB-crystallin (CRYAB) and αA-crystallin (CRYAA) indicates establishment of the lens progenitor-like cells around day 14 of the culture. At this time, the number of PAX6+ and CRYAA+ cells was 65 and 41 %, respectively [122]. Both αA- and αB-crystallins accumulate during the differentiation of lentoid bodies
3-D Cultures of Lentoid Bodies to Improve Their Differentiation Status
A number of potential improvements of the differentiation procedure described above should be considered and empirically tested. In principle, the system can be improved through testing of different 3-D gels and extracellular matrix proteins that are found in the lens capsule, growth of lentoid bodies on lens capsule, specific activators and inhibitors of differentiation, chemical libraries, 3-D scaffolds to generate a gradient of growth factor(s), and any combination of these procedures. In addition, genetically engineered human and mouse ES cells that carry fluorescent reporter genes, under the control of lens regulatory elements, can be used to aid in the analysis of the differentiation process.
There are at least three commercially available 3-D systems: ExtraCel hydrogel (Glycosan Biosystems), HyStem-C Cell Culture Scaffold kit (Sigma), and Cultrex 3-D Culture Matrix Extract (R&D Systems). Each system allows for the incorporation of variable amounts/ratios of laminin, collagen IV, entactin/nidogen, perlecan, fibronectin, collagen XVIII and sparc/osteonectin, extracellular matrix (ECM) proteins found in the lens [21, 117].
A number of drugs have been shown to promote cellular differentiation with some of the tested in lens cell cultures. These include specific inhibitors of DNA methylation such as 5-azacytidine and 5-deazacytidine [12, 49, 94], inhibitors of histone methyltransferases (cytarabine and decitabine [84]), inhibitors of histone deacetylases (valproic acid and sodium butyrate [22, 24, 34, 74, 78]), and inhibitors of cyclin-dependent kinases (olomoucine and roscovitine [70, 73, 89, 115]). Of particular interest are the rho-kinase (ROCK) inhibitors, Y27632 and PP-1, as the PP-1 drug has been successfully used to promote cell cycle withdrawal and commitment of lens cells to differentiate [113, 114].
Considering the specific roles of Notch and Wnt signaling pathways for lens fiber cell differentiation, and the role of Wnt signaling in the differentiation of lens epithelial cells described above (“Mammalian Lens Development and Lessons for a Rational Design of ES Cell-Based Differentiation Systems”), stimulation of ES cell differentiation may be considered. Recombinant Notch ligands, Jagged 1 and 2, can be added transiently during the thirds stage of the differentiation procedure. Concerning Wnt signaling, the situation is more complex as multiple Wnts and their receptors, the frizzled proteins, can regulate lens development both in the epithelial and fiber cell compartments. Nevertheless, inclusion of Wnt3a improved the quantitative parameters of the current procedure of lentoid body formation [122].
Ongoing experiments in the laboratory are aimed to improve differentiation of lentoid bodies using a combinatorial approach as outlined above. The procedure can be improved via genetically engineered ES cells [5] that carry fluorescent markers under the control of lens regulatory regions from genes known to control different stages of the lens lineage formation, cell cycle exit, and terminal differentiation. For this purpose, the EGFP, or enhanced green fluorescent protein marker can be inserted into a specific BAC clone with PAX6 (early marker), HSF4 (late marker), β-/γ-crystallins, DNase IIβ, MIP/aquaporin 0, paralemmin, and other genes expressed in terminally differentiated lens fiber cells as established for similar differentiation systems [83, 110].
iPS Cells and Cataract Research
For the first time in human lens research, we are about to establish a general strategy to model human lens development and diseases with an unlimited supply of lens cells that originate from genetically and phenotypically defined human source(s). In addition, these materials can be shared between multiple laboratories to accelerate research. The pioneering work of S. Yamanaka at the Institute for Frontier Medical Sciences, Kyoto University, Japan, to establish the reprogramming procedure using skin fibroblasts provided proof-of-principle that the iPS cell can be established from somatic terminally differentiated cells, and these iPS cells behaved like authentic ES cells in a series of functional tests [108, 109]. A large follow-up effort in a number of laboratories worldwide resulted in expansion of the reprogramming procedures and cell types suitable for these manipulations. The majority of currently existing procedures are summarized in Table 4.1. It has been shown recently that iPS cells can be produced from a cataract patient using lens epithelial cells as the starting material [85]. Most importantly, these iPS cells were differentiated into lentoid bodies using the procedure described here (see Fig. 4.1) [85]. Nevertheless, whether iPS cells, generated through other reprogramming protocols and cell types, are capable of producing lentoid bodies similar to those generated from human ES cells, remains to be formally proven.
Table 4.1
A representative list of distinct nuclear reprogramming procedures to generate human iPS cells
Starting cell type | Treatment | Abbreviation | References |
---|---|---|---|
Skin fibroblasts | [Oct3/4,Sox2,Klf4,Myc]-retroviruses | iPS | [108] |
IMR90 cells, newborn foreskin fibroblasts | [Oct4,Sox2,Nanog,Lin28]-lentiviruses | iPS | [125] |
Fetal, neonatal, and adult fibroblasts | [Oct4,Sox2,Klf4,Myc]-retroviruses + hTERT + SV40LT | iPS | [81] |
Fibroblasts, liver cells | [Oct4,Sox2,Myc,Klf4]-adenoviruses | Adeno-iPS | [104] |
Terminally differentiated amniotic fluid cells | [Oct4,Sox2,Klf4,Myc]-retroviruses | AF-iPS | [28] |
Amnion-derived cells | [Oct4,Sox2,Nanog]-lentiviruses | hADC-iPS | [127] |
Neural stem cells | [Oct4]-inducible lentivirus | NiPS | [53] |
Peripheral blood mononuclear cells (PB-MNCs) | [Oct4,Sox2,Klf4,Myc]-retroviruses + Htert + SV40LT | BM-iPS | [57] |
Umbilical cord matrix and amniotic membrane | [Oct4,Sox2,Klf4,Myc]-retroviruses, vitamin C, valproic acid | [13] | |
Human newborn fibroblasts (HNFs) | Proteins | [52] | |
Human foreskin fibroblasts | Episomal vector | [124] | |
Human embryonic fibroblasts (HEF) | piggybac transposon | [118] | |
Human peripheral circulating T cells | Sendai virus | TiPS | [95] |
Conclusions and Future Directions
One of the most pressing objectives of medical research today is to develop novel approaches to model formation of human organs, tissues and diseases. Use of human ES and iPS cells differentiated into individual tissues provides the highest possible promise to achieve this objective as it is now possible to understand the contribution of genetic and environmental factors in various diseases including those related to aging such as age-onset cataract.
Thus, the present cell culture system can be used to modulate these common signaling pathways during lens formation [62] via siRNA technology and through the use of small drug molecules, inhibitors of FGF and BMP signaling (e.g., SB431542—an inhibitor of the Alk1 receptor, SU5402—an inhibitor of FGFR and U0126—an inhibitor of MEK) to study formation of lens lineage and formation of alternate cell fates that originate from the common pre-placodal region [105].
It is now possible to produce iPS cells from human patients that carry heterozygous mutations in regulatory genes such as PAX6, FOXE3, MAF, HSF4, PITX3, and others and to identify those genes that are not properly regulated during early stages of lens development. In contrast, studies of cataractogenesis using the system of ES/iPS cells seems be premature until procedures to generate enucleated lentoid bodies with distinct epithelium/fiber cell compartments are established. The long-term benefits of the research to model human cataract using iPS cells should stimulate our efforts to achieve this challenging goal.
Acknowledgements
We thank Dr. Louise Wolf for critical suggestions. We are grateful to Dr. Eric Bouhassira and ES cell core facilities at the Ruth L. and Davis S. Gottesman Institute for Stem Cell Research and Regenerative Medicine of the Albert Einstein College of Medicine for their continuous support. Grant support to AC: R01 EY102200, EY014237 and R21 EY020621. The Department of Ophthalmology and Visual Sciences is supported by an unrestricted grant from Research to Prevent Blindness, Inc.
References
1.
Baker CV, Bronner-Fraser M (2001) Vertebrate cranial placodes I. Embryonic induction. Dev Biol 232(1):1–61PubMed
2.
Balemans W, Van Hul W (2002) Extracellular regulation of BMP signaling in vertebrates: a cocktail of modulators. Dev Biol 250(2):231–250PubMed
3.
Bassnett S (2009) On the mechanism of organelle degradation in the vertebrate lens. Exp Eye Res 88:133–139PubMed
4.
Bassnett S, Shi Y, Vrensen GF (2011) Biological glass: structural determinants of eye lens transparency. Philos Trans R Soc Lond B Biol Sci 366(1568):1250–1264PubMed
5.
Ben-Dor I, Itsykson P, Goldenberg D, Galun E, Reubinoff BE (2006) Lentiviral vector harboring a dual-gene system allow high and homegenous expression in selected polyclonal human embryonic stem cells. Mol Ther 14:255–267PubMed
6.
Benedek GB, Pande J, Thorston GM, Clark JI (1999) Theoretical and experimental basis for the inhibition of cataract. Prog Retin Eye Res 18:391–402PubMed
7.
Bidinost C, Matsumoto M, Chung D, Salem N, Zhang K, Stockton DW, Khoury A, Megarbane A, Bejjani BA, Traboulsi EI (2006) Heterozygous and homozygous mutations in PITX3 in a large Lebanese family with posterior polar cataracts and neurodevelopmental abnormalities. Invest Ophthalmol Vis Sci 47(4):1274–1280PubMed
8.
Blakely EA, Bjornstad KA, Chang PY, McNamara MP, Chang E, Aragon G, Lin SP, Lui G, Polansky JR (2000) Growth and differentiation of human lens epithelial cells in vitro on matrix. Invest Ophthalmol Vis Sci 41(12):3898–3907PubMed
9.
Boswell BA, Overbeek PA, Musil L (2008) Essential role of BMPs in FGF-induced secondary lens fiber cell differentiation. Dev Biol 324:201–212
10.
Brémond-Gignac D, Bitoun P, Reis LM, Copin H, Murray JC, Semina EV (2010) Identification of dominant FOXE3 and PAX6 mutations in patients with congenital cataract and aniridia. Mol Vis 16:1705–1711PubMed
11.
Bu L, Jin Y, Shi Y, Chu R, Ban A, Eiberg H, Andres L, Jiang H, Zheng G, Qian M, Cui B, Xia Y, Liu J, Hu L, Zhao G, Hayden MR, Kong X (2002) Mutant DNA-binding domain of HSF4 is associated with autosomal dominant lamellar and Marner cataract. Nat Genet 31(3):276–278PubMed
12.
Burlacu A (2006) Can 5-azacytidine convert the adult stem cells into cardiomyocytes? A brief overview. Arch Physiol Biochem 112(4–5):260–264PubMed
13.
Cai J, Li W, Su H, Qin D, Yang J, Zhu F, Xu J, He W, Guo X, Labuda K, Peterbauer A, Wolbank S, Zhong M, Li Z, Wu W, So KF, Redl H, Zeng L, Esteban MA, Pei D (2010) Generation of human induced pluripotent stem cells from umbilical cord matrix and amniotic membrane mesenchymal cells. J Biol Chem 285(15):11227–11234PubMed
14.
Chamberlain CG, McAvoy JW (1989) Induction of lens fibre differentiation by acidic and basic fibroblast growth factor (FGF). Growth Factors 1(2):125–134PubMed
15.
Chambers SM, Fasano CA, Papapetrou EP, Tomishima M, Sadelain M, Studer L (2009) Highly efficient neural conversion of human ES and iPS cells by dual inhibition of SMAD signaling. Nat Biotechnol 27:275–280PubMed
16.
Chow RL, Lang RA (2001) Early eye development in vertebrates. Annu Rev Cell Dev Biol 17(255–296)
17.
Churchill A, Graw J (2011) Clinical and experimental advances in congenital and paediatric cataracts. Philos Trans R Soc Lond B Biol Sci 366(1568):1234–1249PubMed
18.
Cvekl A, Duncan MK (2007) Genetic and epigenetic mechanisms of gene regulation during lens development. Prog Retin Eye Res 26(6):555–597PubMed
19.
Cvekl A, Mitton KP (2010) Epigenetic regulatory mechanisms in vertebrate eye development and disease. Heredity 105(1):135–151PubMed
20.
Cvekl A, Piatigorsky J (1996) Lens development and crystallin gene expression: many roles for Pax-6. Bioessays 18(8):621–630PubMed
22.
Davie JR (2003) Inhibition of histone deacetylase activity by butyrate. J Nutr 133(7):2485S–2493SPubMed
23.
Donner AL, Lachke SA, Maas RL (2006) Lens induction in vertebrates: variations on a conserved theme of signaling events. Semin Cell Dev Biol 17(6):676–685PubMed
24.
Duenas-Gonzalez A, Candelaria M, Perez-Plascencia C, Perez-Cardenas E, de la Cruz-Hernandez E, Herrera LA (2008) Valproic acid as epigenetic cancer drug: preclinical, clinical and transcriptional effects on solid tumors. Cancer Treat Rev 34(3):206–222PubMed
< div class='tao-gold-member'>
Only gold members can continue reading. Log In or Register a > to continue
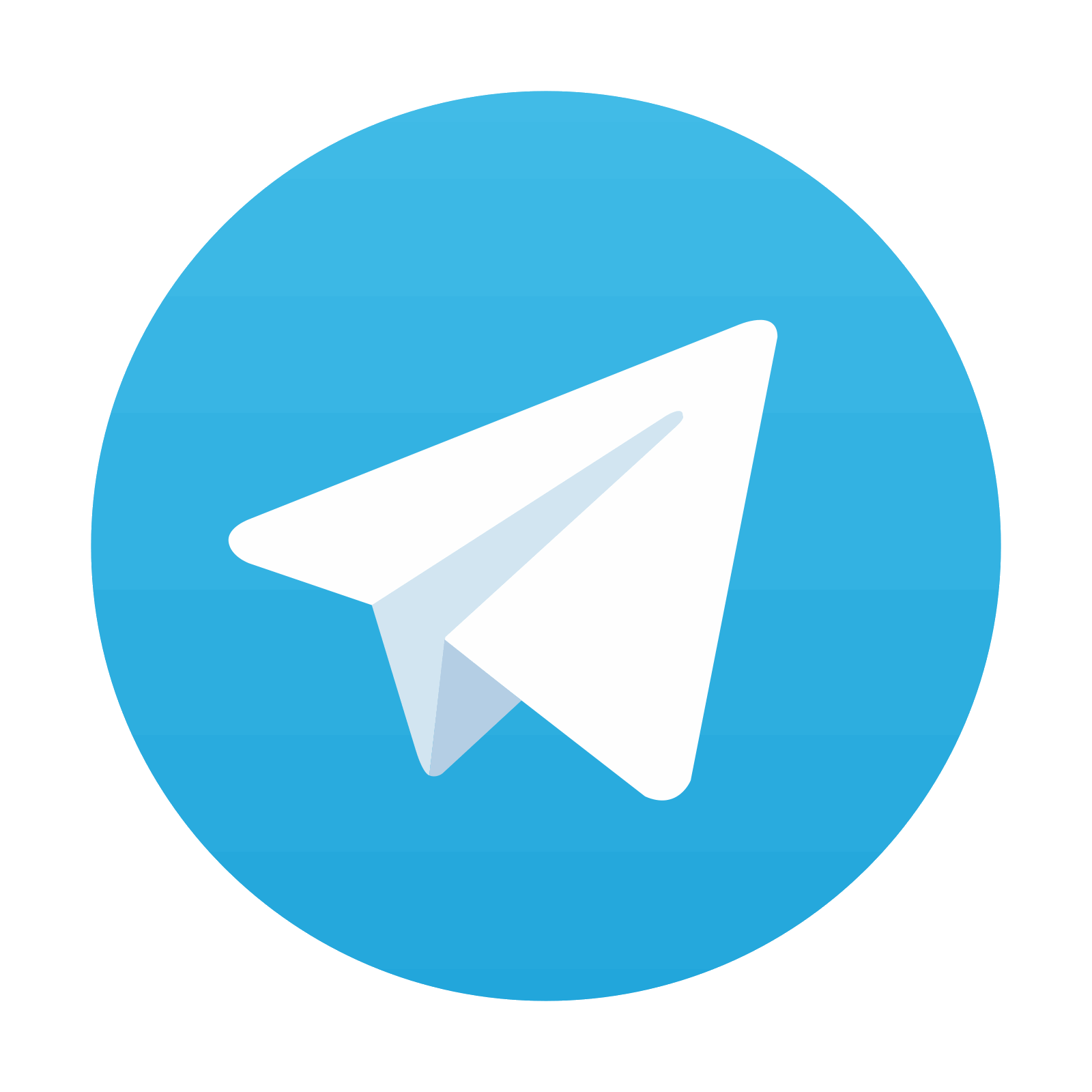
Stay updated, free articles. Join our Telegram channel
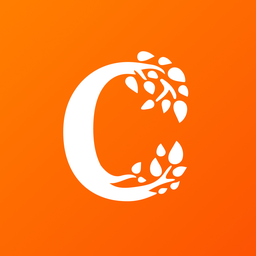
Full access? Get Clinical Tree
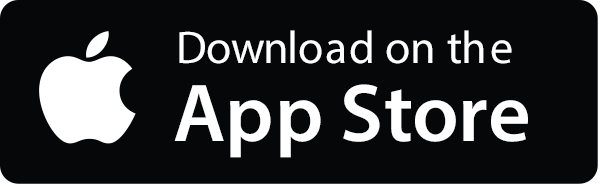
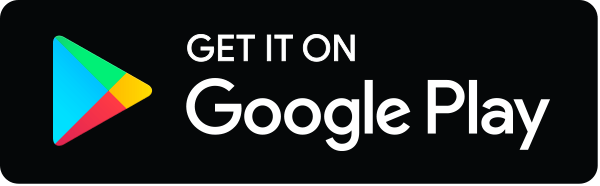