Fig. 63.1
A noncontact, small animal OCT imaging interface with the scan pivot located at the pupil of the murine eye. The imaging interface uses multiple achromatic lenses and a rat eye model based on reference [19]. The collimated beam after the ocular lens is focused on the retina by the optics in the rat eye. For a 1/e 2 beam diameter of 1.4 mm at the cornea, a spot size of ∼15 μm on the retina can be obtained, ignoring aberration from the rat eye. The optical layout was originally generated in the ray-tracing software Zemax and modified
This pivoted scanning configuration has several advantages. First, there is little to no risk of increasing intraocular pressure since there is no cover slip contact to the cornea required. Second, the scanned area on the retina can be more accurately calibrated using an ideal eye model, which is challenging with telecentric scanning due to the unknown pressure applied by the cover slip on the eye. Third, the field of view is limited by the ocular lens diameter as opposed to the pupil in this case, and it is possible to move the field of view to the region of interest simply by adjusting the orientation of the murine eye with respect to the optical axis of the imaging interface. However, these optical designs can be more demanding, and different imaging interfaces are required for imaging different animal species due to the structural differences in the eyes.
63.3 Structural Imaging
Although the murine retina is thinner than the human retina, all major retinal layers observed in the human retina can also be found in the murine retina. For small animal imaging, the optic nerve head can be used as a landmark for alignment and imaging since the murine retina does not have a macula.
63.3.1 Ultrahigh-Resolution Retinal Imaging
Ultrahigh-resolution retinal OCT imaging in animals was first demonstrated in pig and monkey eyes ex vivo in 2003 and 2004 using time domain detection techniques in order to establish a comparison of OCT image features with histological findings [20, 21]. In vivo spectral domain ultrahigh-resolution OCT imaging in the murine retina was demonstrated in 2006 [16, 22]. Ultrahigh-resolution OCT imaging in vivo is challenging because any dispersion mismatch between the sample and reference arms results in a broadening of the axial point spread function. Spectral domain OCT has an advantage that the dispersion mismatch can be numerically compensated by using readily accessible phase information in the spectral OCT signal [23, 24], thereby facilitating ultrahigh-resolution retinal imaging.
Figure 63.2 shows a comparison of a representative histology and ultrahigh-resolution OCT image in a Long-Evans rat [16]. The ultrahigh-resolution system had a bandwidth of 145 nm centered at 890 nm, resulting in an axial resolution of ∼2.8 μm in tissue. The transverse resolution was ∼10 μm in air. The imaging speed was 24,000 A-scans per second. Histology was performed in a sex- and age-matched animal to obtain 7-μm-thick paraffin sections registered to the optic disc. All major retinal layers visible in the human retina can also be observed in the ultrahigh-resolution OCT image of the rat retina with an excellent correspondence to histology. However, a quantitative thickness comparison between histology and OCT images may result in errors since histology tends to generate processing artifacts [20]. The OCT image is displayed in grayscale with brighter pixels corresponding to higher backscattered intensity. Relative backscattered intensities of different intraretinal layers are similar to those observed in the human retina, with the highly backscattering retinal pigment epithelium and relatively less backscattering inner and outer nuclear layers as shown in Fig. 63.2b. It should be noted that the ganglion cell layer cannot be clearly visualized in the OCT image due to speckle and the extremely thin ganglion cell layer thickness in the rat retina.
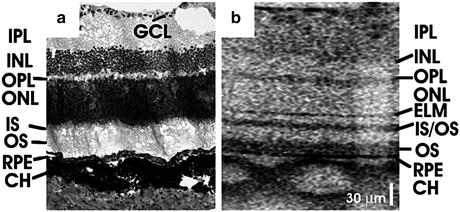
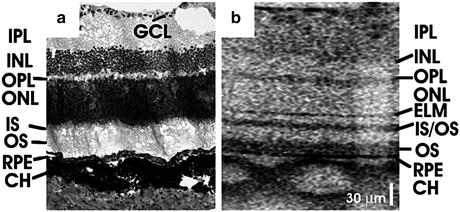
Fig. 63.2
A comparison of a (a) representative histology and (b) ultrahigh-resolution OCT image in a Long-Evans rat retina near the optic nerve head. GCL ganglion cell layer, IPL inner plexiform layer, INL inner nuclear layer, OPL outer plexiform layer, ONL outer nuclear layer, IS photoreceptor inner segment, OS photoreceptor outer segment, RPE retinal pigment epithelium, CH choroid, ELM external limiting membrane, IS/OS photoreceptor inner and outer segment junction. Scale bar: 30 μm (Image reproduced from Srinivasan et al. [16])
Figure 63.3 shows a representative ultrahigh-resolution OCT image from a normal C57BL6 mouse acquired with the same ultrahigh-resolution OCT system [16]. All major intraretinal layers except the ganglion cell layer can be clearly visualized in the mouse retina with ultrahigh-resolution OCT.
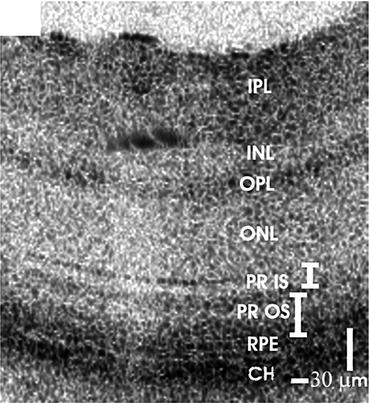
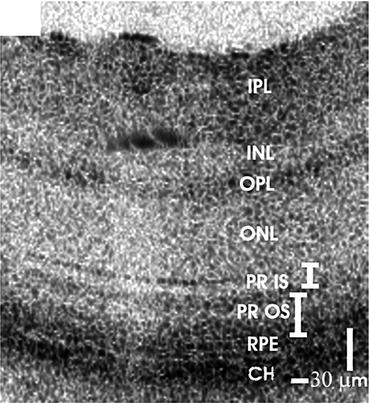
Fig. 63.3
A representative ultrahigh-resolution OCT image from a normal C57BL6 mouse. IPL inner plexiform layer, INL inner nuclear layer, OPL outer plexiform layer, ONL outer nuclear layer, PR IS photoreceptor inner segment, PR OS photoreceptor outer segment, RPE retinal pigment epithelium, CH choroid (Image reproduced from Srinivasan et al. [16])
Figure 63.4 shows an example application of ultrahigh-resolution OCT technology in a rat model of retinal disease using the same OCT system as in Figs. 63.2 and 63.3 [11]. Using ultrahigh-resolution OCT, it was possible to observe retinal edema produced by intravitreal injection of carbonic anhydrase-I, an intracellular enzyme identified in the proliferative diabetic retinopathy vitreous proteome, in a streptozotocin-induced diabetic rat model. The identification of structural changes in early disease or processes such as vascular permeability changes is important because these can serve as surrogate markers for investigating the molecular pathways of disease for the development of new pharmaceuticals.
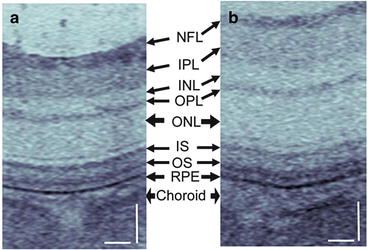
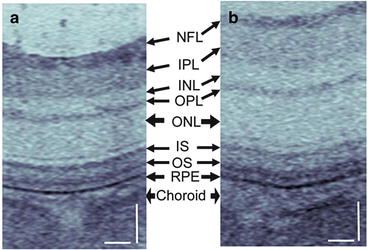
Fig. 63.4
Ultrahigh-resolution OCT can visualize changes in intraretinal layer thickness produced by intravitreal injection of (a) saline vehicle control versus (b) carbonic anhydrase-I in streptozotocin-induced diabetic rats. Scale bar: 50 μm (Image reproduced from Gao et al. [11])
In addition to increasing the axial resolution using ultrahigh-resolution OCT, it is also possible to increase the transverse resolution in small animal retinal imaging using adaptive optics. Since the numerical aperture available in the murine eye is larger than that in the human eye, the maximum theoretically achievable transverse resolution on the retina with adaptive optics is higher in small animals than in humans [25, 26]. Therefore, combining ultrahigh-resolution OCT and adaptive optics would enable ultrahigh-resolution imaging of the small animal retina in all three dimensions. However, visualization of tissue at the cellular level not only requires ultrahigh axial and transverse resolutions but also high enough contrast between different subcellular organelles, which in histology is provided by difference in staining properties. Although current ultrahigh-resolution OCT technology does not yet enable cellular level visualization, it is still possible to detect morphologic alternations in the intraretinal structure due to retinal diseases, which is a powerful advantage over histology.
63.3.2 Ultrahigh-Speed Retinal Imaging
Ultrahigh-resolution spectral domain OCT system design is more challenging than standard resolution system design because of the broader bandwidth required for ultrahigh axial resolution. Therefore, many commercial OCT systems currently offer axial resolutions of 5–7 μm in tissue. Recent advances in CCD and CMOS camera technology enabled ultrahigh-speed spectral domain OCT with an imaging speed >200 kHz A-scan rate with a system complexity comparable to commercial spectral OCT systems at slower imaging speeds [27]. While ultrahigh-resolution OCT provides a higher axial resolution and inherently smaller speckle size, ultrahigh-speed imaging enables spatial compounding and image averaging methods to enhance visualization of intraretinal layers in small animals by reducing the effects of speckle.
Figure 63.5 shows OCT images from a normal Sprague Dawley rat. The ultrahigh-speed spectral domain OCT system had an imaging speed of 244,000 A scans per second. The bandwidth of the light source was ∼55 nm centered at 840 nm, yielding an axial resolution of 5.7 μm in tissue. The theoretical spot size on the retina using a standard rat eye model was ∼15 μm. While Fig. 63.5a shows a single OCT B-scan consisting of 700 A-scans, Fig. 63.5b shows an average of six neighboring B-scans uniformly distributed in a thin strip ∼13-μm thick. Note that speckle is dramatically suppressed by averaging multiple neighboring B-scans due to spatial compounding. This comes at the cost of slight reduction in the effective transverse resolution, but enhancement in image quality due to speckle suppression and increased signal-to-noise ratio outweighs the trade-off for most applications. Because Sprague Dawley rats have significantly less pigment in the retinal pigment epithelium and choroid than in Long-Evans rats, OCT images of the Sprague Dawley retina can visualize down to the sclera, which can be useful for applications where choroidal or scleral abnormalities are expected.
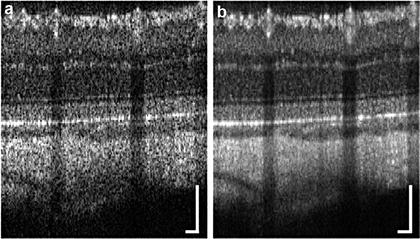
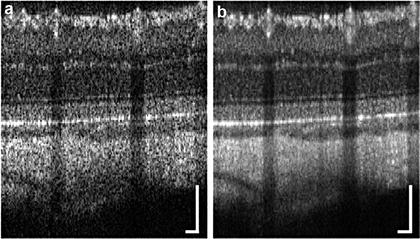
Fig. 63.5
Ultrahigh-speed OCT images extracted from a volumetric data set (a) before averaging and (b) after averaging six neighboring OCT B-scans. The volumetric data set consisting of 700 × 700 A-scans was acquired from a 1.5 × 1.5-mm2 area centered at the optic nerve head in a Sprague Dawley rat. The effect of averaging can be clearly seen in terms of speckle reduction and increase in signal-to-noise ratio, which greatly enhances visualization of intraretinal layers. Scale bar: 100 μm
It should be noted that ultrahigh resolution and ultrahigh speed are not fundamentally incompatible in spectral domain and swept source OCT. With advances in OCT technology, it will be possible to achieve ultrahigh imaging speeds and ultrahigh axial resolutions simultaneously, thereby combining the advantages of the two techniques in a single OCT system.
63.3.3 Long Wavelength Imaging
In human retinal imaging, absorption of light by water in the anterior eye and vitreous limits the usable wavelength range. Therefore, human retinal OCT imaging typically uses near-infrared (NIR) wavelengths shorter than 900 nm or around 1,060 nm, where absorption of light is negligible or within a transmission window [28]. Unlike wavelengths shorter than 900 nm where ultrahigh-resolution retinal imaging with a broadband NIR light source is feasible, wavelengths around 1,060 nm are at a local minimum in water absorption which has a relatively narrow bandwidth [29], which makes achieving an axial resolution <5 μm in the human retina challenging regardless of the light source bandwidth. In addition human retinal OCT imaging at wavelengths >1,150 nm is impossible since almost all light is absorbed by the anterior eye and vitreous.
The axial eye length is ∼6.1 mm in the rat and ∼3.3 mm in the mouse [17, 18], considerably shorter than the axial eye length of ∼24 mm in humans. According to the Beer-Lambert law, due to the shorter axial eye lengths, water absorption will be considerably less severe in the murine eye than in the human eye as shown in Fig. 63.6. This implies that retinal OCT imaging using longer wavelengths can be achieved in murine eyes. In both rat and mouse eyes, the water absorption window at ∼1,060 nm does not have the same bandwidth limiting effects as in the human eye, making ultrahigh-resolution retinal imaging feasible at these wavelengths in the murine eye. Furthermore, there is a significant transmission through the ocular media even at ∼1,300 nm wavelength. Multiple studies have investigated long wavelengths for retinal OCT imaging in the murine eye. The first demonstration of retinal imaging in the mouse eye using time domain OCT utilized wavelengths centered at ∼1,280 nm [10]. Spectral domain ultrahigh-resolution retinal imaging at ∼1,060 nm wavelengths with an axial resolution <3 μm was demonstrated in the rat eye [30]. Retinal imaging at ∼1,310 nm was demonstrated in the rat eye using spectral domain OCT [31].
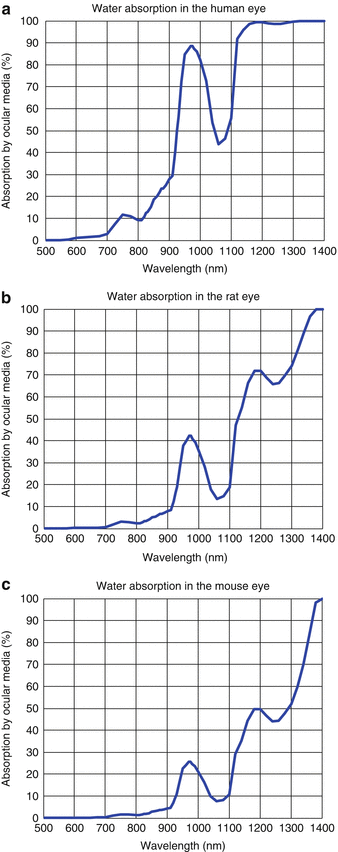
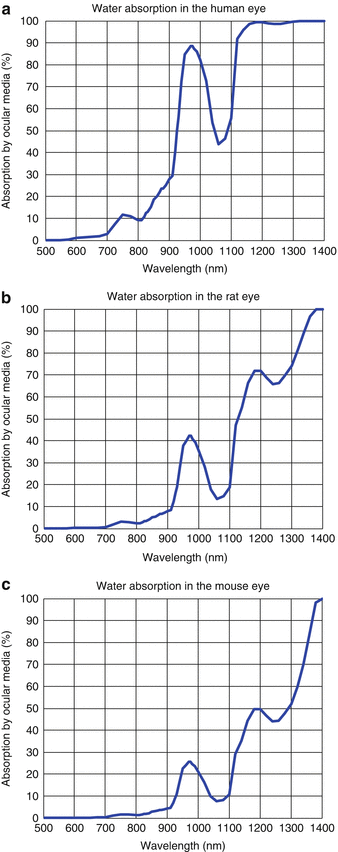
Fig. 63.6
Absorption of light by water as a function of wavelength in (a) the human eye, (b) the rat eye, and (c) the mouse eye. Axial eye lengths of 24, 6.1, and 3.3 mm were assumed for the human, rat, and mouse eyes, respectively. Water absorption coefficients were obtained from Ref. [28]
Using longer wavelengths for small animal OCT imaging may have advantages in small animal models of ocular diseases that develop cataracts and/or other forms of ocular opacity such as vitreous hemorrhage or choroidal neovascularization. Longer wavelengths have reduced scattering, and better visualization of the retina and choroid has been demonstrated in human subjects and patients with cataracts [32, 33]. Ocular opacities caused by blood are also expected to affect the OCT image quality less at longer wavelengths [34].
63.3.4 Whole Eye Imaging
Whole eye imaging using Fourier domain OCT is significantly easier in murine eyes than in human eyes due to the relatively short axial eye lengths in small animals. Although time domain OCT can also be used for whole eye imaging in small animals [35], Fourier domain OCT such as spectral domain or swept source OCT has the advantage that imaging can be performed orders of magnitude faster due to its inherently higher sensitivity. Faster imaging speeds at a given signal-to-noise ratio are advantageous since image averaging can be performed in order to reduce the effects of speckle and increase image quality. Because the numerical aperture of the imaging interface is limited for small animal whole eye imaging due to the relatively long depth of focus required, the signal-to-noise ratio tends to be relatively low for whole eye imaging. Therefore, higher quality images provided by image averaging is essential for better layer visualization and more accurate biometry. Multiple studies have demonstrated whole eye imaging in the mouse and rat eyes using swept source OCT [36–38].
An example of long wavelength ophthalmic OCT imaging in small animals is shown in Fig. 63.7 [37]. The images were acquired with a swept source OCT system using 1,060-nm wavelengths with an imaging speed of 28,000 A-scans per second. The imaging interface was using the telecentric scanning configuration. In order to achieve a sufficient imaging range, a full range OCT imaging algorithm was used, resulting in a total imaging range of 6 mm [39]. High-quality OCT images of mouse eyes were acquired by averaging 50 OCT B-scans with 512 A-scans each. It should be noted that with telecentric scanning, the effective transverse scan range on the retina is essentially limited to a single spot due to optical refraction from the anterior eye. This effect is clearly seen after refractive correction during post-processing as shown in Fig. 63.7b. Regardless, biometric parameters such as axial lengths and radii of curvature of the cornea, anterior chamber, lens, and vitreous can be extracted using whole eye OCT imaging.
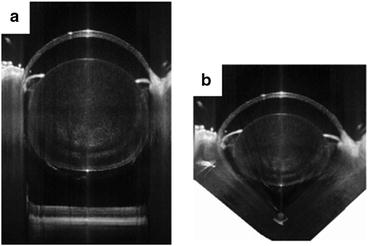
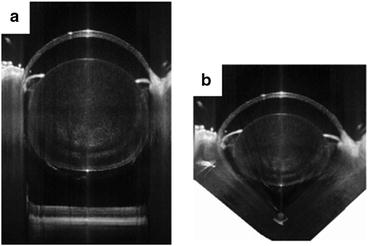
Fig. 63.7
An OCT image of a whole eye of a MF1 mouse (a) before refraction correction and (b) after refraction correction (Image reproduced from Wang et al. [37])
63.4 Functional Imaging
In addition to structural imaging, OCT can also be used for functional imaging in the murine retina. In this section, functional imaging techniques such as measurements of intraretinal layer reflectivity in response to light stimuli, retinal blood flow measurements using Doppler OCT, OCT angiography imaging of the retinal capillary network, and spectroscopic OCT imaging will be discussed. Functional imaging can be more demanding than simple structural imaging because it typically requires differential measurements. Ultrahigh-resolution physiology measurements need to detect differential changes in intraretinal layer reflectivities. Doppler OCT requires time differential measurements in the OCT phase. OCT angiography of the retinal capillary network requires mapping of intensity and/or phase changes in time. Spectroscopic OCT measures differential absorption at different wavelengths.
63.4.1 Ultrahigh-Resolution Physiology Measurement
Intrinsic signals from biological tissue detected with optical imaging techniques can be used for measuring neural activity. In the brain, cortical activity can be mapped by imaging intrinsic signals using optical techniques [40]. In the retina, changes in intrinsic optical signals in response to light stimuli have been detected using fundus reflectometry [41, 42]. Although different imaging techniques may measure different types of intrinsic signals, OCT has the advantage that it can detect depth-resolved changes in optical properties of intraretinal layers in response to visual light stimulus. Using time domain OCT, measurement of changes in optical scattering properties activated by visible light was demonstrated ex vivo in the isolated retinae of the frog and rabbit [43, 44]. Figure 63.8 shows an example of ex vivo measurements of backscattered reflectance in the rabbit retina using time domain OCT [44]. As shown in Fig. 63.8d, e, it can be seen that the backscattered reflectance changes in different intraretinal layers due to the visual stimulus.
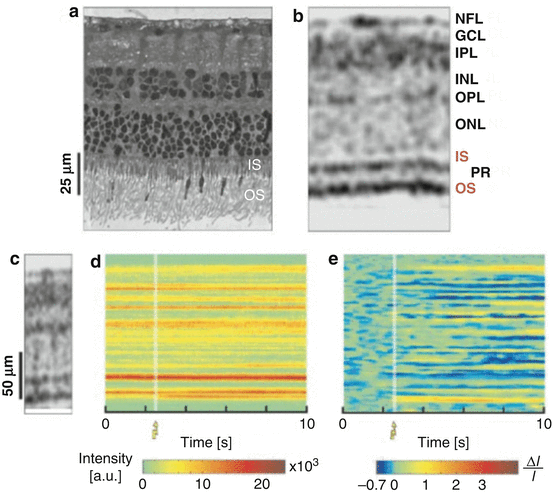
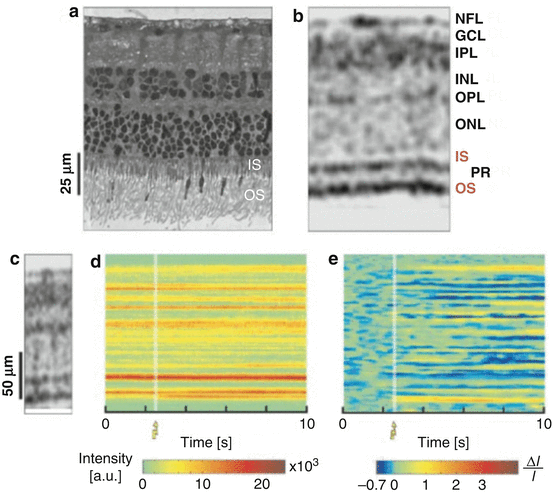
Fig. 63.8
(a) A histologic cross section of the rabbit retina compared with (b) an ultrahigh-resolution time domain OCT image. (c) An example of OCT A-scans repeatedly acquired from the same position on the retina. (d) OCT A-scans acquired from the same position before and after visual stimulus. (e) Differential OCT A-scans calculated from (d) by taking the ratio of the changes in signal intensity relative to baseline and the signal intensity at baseline. The white bar shows the onset and duration of the visual stimulus (Image reproduced from Bizheva et al. [44])
However, because OCT is a noninvasive imaging technique, it can also be used for in vivo functional measurement of changes in scattering properties of intraretinal layers. In vivo OCT measurement of intrinsic optical signals is challenging because of the small layer thicknesses, speckle, and eye motion. Speckle produces a random spatial fluctuation in backscattered light intensity, which makes quantitation of backscattered reflectivity challenging. Eye motion makes imaging the same location on the retina as a function of time difficult and also introduces noise due to changes in speckle with parasitic eye motion. Ultrahigh-resolution OCT imaging has an advantage that the multilayered retinal structure can be resolved with a <3-μm axial resolution. Although the transverse resolution is not as fine as the axial resolution for OCT without adaptive optics, the spatial variation in the retina in the transverse direction is typically significantly less than that in the axial direction. High-speed imaging with spectral domain OCT (as opposed to time domain OCT) enables novel scanning and signal averaging schemes which can reduce the effects of speckle and eye motion.
In vivo measurements of functional changes in intrinsic optical signal were first demonstrated in the rat retina using ultrahigh-resolution spectral domain OCT [22]. Measurements were performed in Long-Evans rats, using a high-speed ultrahigh-resolution spectral domain OCT system at 24,000 axial scans per second with a 2.8-μm axial image resolution. Animals were dark adapted before measurements were taken. Because the OCT light source was in the NIR wavelength range, the OCT beam was not visible to the animals. In order to compensate eye motion and reduce speckle, a small area of 160 × 160 μm2 was repeatedly scanned on the retina at a 6.2-Hz volume acquisition rate. Eye motion in the transverse direction was corrected by cross-correlating sequential volumes, and A-scans were aligned in the axial direction with respect to the photoreceptor inner and outer segment junction.
Figure 63.9 shows the results of ultrahigh-resolution functional physiology measurements. Figure 63.9a shows the percent change in amplitude reflectance as a function of imaging depth before and after applying a light stimulus on the rat retina with respect to a baseline measurement. Note that the amplitude reflectance was calculated by averaging all A-scans from a single volume after correcting for transverse and axial eye motions in order to reduce speckle. The stimulus resulted in a maximum increase of ∼12 % in backscattered amplitude reflectance. Figure 63.9b, which shows all A-scans from a single OCT volume for comparison, indicates that the increase in reflectance was primarily localized to the photoreceptor outer segment.
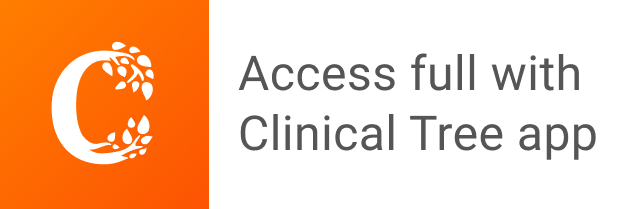