CHAPTER 79 Skin Flap Physiology and Wound Healing
An important concept in skin vascular physiology as it applies to flaps is that of the angiosome. In 1987, Taylor and Palmer1 coined the term angiosome to refer to the tissue supplied by a named artery. The head and neck region has several such angiosomes, including the important thyroid, facial, buccal, ophthalmic, superficial temporal, and occipital.1 Adjacent angiosomes are in communication with each other via choke vessels. This relationship becomes important in the design of various flaps. It has been demonstrated that axial pattern flaps (to be discussed in this chapter) are capable of supplying adequate blood flow to an adjacent angiosome. The incorporation of tissue on an additional angiosome beyond this adjacent angiosome, however, invites vascular compromise and likely flap failure. Techniques to extend this vascular supply, such as delay, may be necessary.
Zones of Perfusion
Maintenance of cell function and viability is the objective of the circulatory system at macrocirculatory, capillary, interstitial, and cellular levels. The importance of tissue vascularity is well recognized, but the complexity and diversity of tissue perfusion mechanisms require that the process be divided into components. Each level of perfusion has unique physiologic characteristics, and optimal management is possible only if these differences are recognized. Johnson and Barker2 described two levels of risk zones for flap failure, making a distinction between thrombosis at the arterial and capillary levels. By extension of this concept, four zones of the circulatory system can be considered for all hazards (Fig. 79-1). Proper function of each zone is crucial to tissue viability.
Zone I consists of the cardiopulmonary system, the conduits for blood flow (arteries and veins), neural control of those conduits, and the lymphatic system. Zone I has historically been recognized as essential for flap survival. The delay phenomenon is primarily a zone I effect. The development of the pedicled flap3 underscores the importance of zone I in extending flap survival time. Free microvascular tissue transfer is also a zone I manipulation.
Zone II comprises the capillary circulation. The importance of the capillary circulation is shown by the “no-reflow phenomenon,” wherein a loss of nutritive blood flow occurs in the presence of an adequate vascular supply.4
Zone III is the interstitial space and its mechanisms of nutrient delivery. The capillary wall is included in zone III because capillary permeability is a main determinant of interstitial space properties. Failure of metabolites to enter and traverse the interstitial space can result in loss of cell viability, even though there is adequate zone I and even zone II function. Interstitial systems are an important link in skin flap survival rate.5
Anatomy and Physiology of Skin
The epidermis of the skin is derived from ectoderm in the early embryo. The glandular appendages of the skin (i.e., sebaceous glands and hair follicles) develop from tubes and solid cords that invaginate from the covering ectoderm.6,7 The epidermis is a metabolically active, but avascular, stratified squamous epithelium. The average epidermal thickness for most of the body is 0.1 mm. The majority of cells undergo keratinization and form the various epithelial layers.8 The superficial keratinized cells of the skin are continuously replaced by cells originating from the mitotic activity in the basal layer of the epidermis. Melanocytes derived from neural crest cells also are found in the epithelium of skin and constitute a second cell type.
The dermis, derived from embryonic mesoderm, consists primarily of noncellular connective tissue. This relatively noncellular layer has metabolic requirements far less than those of the epidermis. Nerves, blood vessels, lymph vessels, and the base of the epidermal glandular appendages are found within the dermis.8 The dermis is 15 to 40 times thicker than the epidermis, with a maximum width of 4 mm on the back.9 The outer surface of the dermis has an uneven border contacting the epidermis that is known as the papillary layer. The papillary dermis is characterized by an abundant ground substance, irregularly arranged collagen bundles, and a highly developed microcirculation. The reticular dermis is composed of thick bundles of collagen and coarse elastic fibers, and fibrocytes and blood vessels are proportionally less numerous.
Zone I: Macrocirculatory System
Blood vessels travel by one of two main routes to terminate in the cutaneous circulation. Musculocutaneous arteries pass through the overlying muscle to which they provide nutrition; septocutaneous arteries10 (also referred to as direct cutaneous arteries) travel through fascial septa that divide the muscular segments (Fig. 79-2).
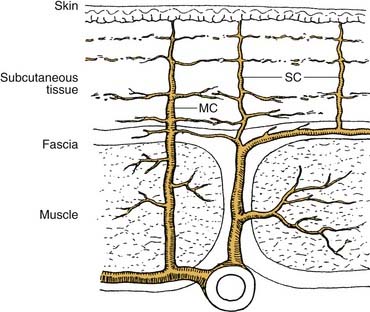
Figure 79-2. Varying pathways to skin define musculocutaneous (MC) and septocutaneous (SC) arteries.
The cutaneous portion of a septocutaneous artery typically runs parallel to the skin surface, providing nutrition to a large area of skin. Septocutaneous arteries are usually accompanied by pairs of veins and run above the superficial muscular fascia.11 The more common musculocutaneous arteries leave the muscle and enter the subcutaneous tissue to supply a smaller region of skin.
Septocutaneous and musculocutaneous arteries empty into a diffuse interconnecting vascular network of dermal and subdermal plexuses. This network provides a redundancy in the vascular supply to the skin. A collateral blood supply supports the vascular territory of each artery. Lymphatic vessels form a plexus running parallel and deep to the network of blood capillaries. The lymphatic capillaries, which end in blind sacs, conduct extracellular fluid back into the blood stream. Lymphatic function is affected by inflammation and loss of blood vessel pulsations.8
The neural supply to the skin originates from sensory nerves and sympathetic nerves. The sensory nerves are distributed in segmental fashion, forming dermatomes, and participate in the skin’s protective function. The postganglionic terminals of cutaneous sympathetic nerves contain the neurotransmitter norepinephrine and are found in the area of cutaneous arterioles.12
Zone II: Capillary System
The cutaneous capillary system, along with the arteriovenous shunts, serves the two important functions of nutritional support and thermoregulation. Primarily because of its thermoregulatory function, the rate of blood flow through the skin is one of the most variable in the body. During ordinary skin temperatures, the amount of blood flowing through the skin (approximately 9 mL/min per 100 g of tissue) is greater than the flow required for nutritional support.13,14 Blood flow can increase to 20 times this value with maximal vasodilation. When the body is exposed to extreme cold, blood flow can be reduced to levels that are marginal for cutaneous nutrition.
Before entering the capillary bed, the arterioles branch into small vessels (e.g., terminal arterioles or metarterioles) that are surrounded by a discontinuous layer of smooth muscle. A simple ring of smooth muscle forms a sphincter at the point where the capillaries originate from the metarteriole. This sphincter can completely stop blood flow within the capillary. The capillary bed can be bypassed by arteriovenous shunts that allow the arterioles to empty directly into venules. During conditions of adequate systemic vascular pressure, preshunt and precapillary sphincters regulate the distribution of cutaneous blood flow.16
The preshunt sphincters are involved in regulating the changes in blood flow that affect thermoregulation and systemic blood pressure.16,17 Release of norepinephrine by the postganglionic sympathetic fibers results in contraction of the preshunt sphincters. This contraction diverts blood away from the skin surface, where heat loss could occur. With increased body temperature, the sympathetic vasoconstrictor impulses decrease, allowing for greater blood flow to the skin.
Local production of bradykinin may play a role in regulating skin blood flow by causing vasodilation.15 The cutaneous circulation also is extremely sensitive to circulating norepinephrine and epinephrine. Even in areas of skin that have lost their sympathetic innervation, a mass discharge of the sympathetic system still results in intense vasoconstriction in the skin.
The precapillary sphincter, which controls the amount of nutritive blood flow to the skin, responds to local hypoxemia and increased metabolic byproducts by dilating.16–18 Capillary blood flow is also affected by elevated interstitial pressure, which can compress the capillary and decrease transcapillary flow. Conversely, as pressure decreases in the interstitium, the capillary expands and flow increases.19 Acute rises in systemic blood pressure cause an immediate increase in local blood flow followed by return of flow to near normal levels in less than 1 minute. This normalization of capillary blood flow is called autoregulation.20 The metabolic theory for autoregulation proposes that an excess of oxygen and nutrients results in arteriolar constriction. This process is believed to be the primary functional mechanism of autoregulation. The myogenic theory suggests that stretching of the arteriolar muscle results in reflex contraction and may be a mechanism to protect capillaries from excessively high blood pressures. Reduction of blood flow may also occur as a result of increased interstitial pressure.
Zone III: Interstitial System
The interstitial space is filled with proteoglycans and collagen.13 In many tissues, hyaluronic acid filaments make up the interstitial ground substance. These filaments are normally woven through the interstitium, producing a medium that exhibits high resistance to fluid movement unless the tissue is well hydrated. In tissues with excess edema, the filaments do not overlap, producing shear planes of free fluid within the interstitium.5
Two processes by which substances can move across the interstitial space are diffusion and convection.21,22 Diffusion is the process wherein a molecule passively moves to a lower concentration. Molecular movement by diffusion is affected by many factors. An important characteristic is lipid solubility. Lipid-soluble molecules tend to traverse cell membranes more easily and quickly than non–lipid-soluble agents. Large molecules diffuse more slowly than small ones. As molecules diffuse away from the capillary, the concentration of that molecule decreases by the inverse square law. As edema occurs, diffusion distances increase.23
Convective flow of nutrient-laden plasma out of the capillaries and across the interstitial space is produced by a combination of hydrostatic and osmotic pressures as described by Starling in 1896.24 The equation is as follows:
A net change of 2 mOsm is sufficient to increase interstitial pressure beyond transmural capillary pressure, thereby compressing the capillary. Increased venous pressure limits interstitial resorption of fluid and contributes to formation of an interstitial transudate.25 Inflammation in a flap is probably a result of nociceptor pathway stimulation by handling of the flap. Even the relatively minor trauma of inserting a fine needle causes a “very large” increase in capillary permeability within minutes after insertion, as evidenced by rapid influx of proteins into the interstitium.26 Ischemia also produces inflammation. Capillary hyperpermeability has been found throughout ischemic flaps.27,28 The lymphatic system has two functions that affect zone III, to remove excess fluid and to remove interstitial protein. There is some controversy in the physiology literature regarding the magnitude of the latter function.21 If the lymphatic system is malfunctioning, interstitial fluid and interstitial protein accumulate.29,30
Zone IV: Cellular Systems
The intracellular space is the endpoint for nutrient transport and the origin of metabolic waste. The cell wall is a fluid lipid bilayer 7.5 to 10.0 nm thick. The lipid bilayer is a major barrier to movement of solutes across the membrane. The primary function of the cell membrane is to maintain or to vary in a controlled fashion the separation of the extracellular and intracellular environments. This separation is achieved by specific membrane proteins, which act as solute pumps and solute and solvent leaks. The osmotic pressure between the two environments also should be maintained to preserve normal cell volume. The sodium-potassium pump is important in maintaining osmotic equilibrium across the cell membrane and has a requirement for energy in the form of adenosine triphosphate (ATP).31 A loss of energy substrate (oxygen and consequently ATP) produces an intracellular movement of sodium and an increase in intracellular osmotic pressure.25 These changes occur quickly,32 within 10 minutes after arterial occlusion. Within seconds of hypoxia, levels of ATP begin to decrease, and cells begin to swell.29 Relatively brief periods of ischemia result in a reversible swelling of the cell and organelles. If the ischemic insult is severe and prolonged, cell lysis and flap necrosis occur.
Classification of Flaps
Flaps are commonly classified according to their principal blood supply. They include random, arterial cutaneous (i.e., axial), fasciocutaneous, musculocutaneous, and venous flaps (Fig. 79-3). Venous flaps to this point have had no application in head and neck reconstruction. Flaps may also be described according to site of origin relative to the area to be reconstructed. Thus, flaps utilized in the head and neck are local, regional, or distant. Distant flaps may be pedicled or free flaps (microvascular free tissue transfer).
Myocutaneous and Fasciocutaneous Flaps
The greater blood flow and higher tissue oxygen tensions available with myocutaneous flaps33 make this design superior in the management of contaminated or infected defects.34,35 Improved phagocytotic and bactericidal activity of leukocytes is seen in myocutaneous flaps in comparison with random pattern flaps in the canine model.36 These physiologic benefits contribute to the ability of myocutaneous flaps to resist bacterial inoculation more effectively than random pattern flaps.
Fasciocutaneous flaps use direct arterial (septocutaneous) vessels with the cutaneous branches at the level of the deep fascia forming a plexus, which supplies the subdermal plexus.37 The appropriate size of fasciocutaneous flaps is less well defined than axial pattern flaps with their obvious arterial supply.38 Fasciocutaneous flaps appear to rely more on potential skin vascular territories. Four types of fasciocutaneous flaps have been described on the basis of the pattern of blood supply incorporated into the fascial component of the flap. Examples are the parascapular flap and the radial forearm flap.39,40
Physiology of Acutely Raised Flaps
Impairment of Vascular Supply
Partial interruption of the vascular supply (zone I) to the skin is the most obvious and critical change that occurs with elevation of a cutaneous flap. Flap survival depends on the severity of ischemia and the amount of time before recovery of nutrient blood flow. Flaps can tolerate and survive an average of 13 hours of complete avascularity.41 Limitation of the vascular supply results in a local decrease in perfusion pressure to the skin. In arterial or myocutaneous flaps, the blood supply to the skin overlying the vascular pedicle is usually adequate.42 In random flaps or random extensions of flaps, the decrease in perfusion pressure becomes more pronounced with increasing distance from the base of the flap.43,44 When perfusion is reduced in one area of a random flap, the adjacent vascular territories supplied by a separate perforating vessel can provide a low-pressure blood supply through the subdermal plexus (Fig. 79-4). Because the nutritional requirements of skin are relatively low, a number of vascular territories can be compromised before necrosis results.
The surviving length of the random portion of the flap depends on the physical properties of the supplying vessels (intravascular resistance) and the perfusion pressure.45,46 When the perfusion pressure drops below the pressure in the interstitial space, capillary blood flow ceases. The pressure at which there is no longer enough intravascular blood pressure to maintain capillary blood flow is called the critical closing pressure.
In the past, random cutaneous flaps often were designed according to a desired length-to-width ratio, a wider base being needed to successfully transfer a longer flap. The wider random flap includes only additional vessels with the same perfusion pressure. The relationship between perfusion pressure and critical closing pressure is not altered, and there is no change in survival length (Fig. 79-5).9,47
In free flaps, a secondary ischemia can occur when thrombus forms at the anastomosis. Anastomosis failure is related to exposure of the vascular intima to platelets.48 Platelet activation is caused by superficially exposed subendothelium or, with deeper vessel injury, exposure to collagen types I and III. The geometry of the vascular arrangement is often a critical factor when experienced surgeons lose a free flap., Measurements of transcutaneous oxygen pressure (TcPO2) and transcutaneous carbon dioxide pressure (TcPCO2) have been investigated for monitoring of compromised flaps. In experimental studies TcPO2 was found to be more sensitive to flap ischemia; however, in clinical studies it was difficult to distinguish between flap ischemia and congestion on the basis of TcPO2 alone. Instead, a TcPCO2 greater than 90 mm Hg in a congested flap proved a more useful guideline for warranting further treatment.49
The venous outflow from the skin also is impaired with flap elevation. Venous flow can occur through the subdermal plexus or via venous channels that accompany the feeding artery in the pedicle. Complete venous occlusion in the early postelevation period may be more damaging to flap survival than inadequate arterial supply.48 Fortunately, the subdermal plexus alone is often able to provide adequate venous outflow. Care should be taken to preserve venous outflow in flaps pedicled solely on the feeding vessels.
Impairment of lymphatic drainage with flap elevation also occurs. Reduction of the cutaneous lymphatic drainage results in a rise in interstitial fluid pressure that is compounded by increased leakage of intravascular protein associated with inflammation. The resulting edema leads to increased interstitial pressure, which decreases capillary perfusion by raising the critical closing pressure (Fig. 79-6).24,45 Alterations in the Starling forces result in further ischemic swelling of cells and the interstitial space, setting a positive feedback circle in motion.
Microcirculatory Changes
Necrosis in flaps is caused by prolonged loss of nutritional blood flow.50,51 There are several proposed mechanisms for loss of nutritional flow, each of which may play a role. Erythrocyte sludging has frequently been noted in capillaries undergoing ischemia, often without thrombus formation. Therefore, sludging is not necessarily caused by platelet activation unless a microvascular anastomosis or other vessel injury is involved, such as tearing, stretching, laceration, or crushing. Microemboli are commonly generated from a microvascular anastomosis.52
The reason for the erythrocyte sludging is multifactorial. Erythrocytes become turgid and lose their flaccid, biconcave disk shape in the acidic environment of ischemia. Narrowing of the capillaries,9,53 presumably a result of external compression, also contributes to stacking of erythrocytes within the lumen of ischemic tissue capillaries. Sludging is seen less often if the hematocrit value is kept below 30%.54
Capillary blood flow is further hindered by leukocyte adherence to the capillary wall.55 The leukocytes are probably responding to cytokines released from the cells in response to initial injury. The leukocytes first roll along the capillary wall and then begin to stick. As time progresses, the leukocytes flatten onto the endothelial cell and eventually pass into the interstitium. Each step of the process has biochemical mediators. In patients undergoing heart transplantation, leukocyte depletion decreases ultrastructural evidence of reperfusion injury.56 Ischemic cells of all tissues swell because of the loss of energy substrate needed to continue maintaining the osmotic gradient. Swelling of extravascular cells causes compression of the vascular lumen,57 but endothelial cell swelling has a greater effect on capillary blood flow.58 Histologic evidence of edema can be used to grade the severity of free flap injury.59 Nitric oxide synthase, an endothelium-derived vasodilator, has been studied for its effect in skin flaps.60–64
Neovascularization
In surviving flaps, blood flow gradually increases. If the flap is in a favorable recipient site, a fibrin layer forms within the first 2 days. Neovascularization of the flap begins 3 or 4 days after flap transposition.65,66 Revascularization adequate for division of the flap pedicle has been found as early as 7 days in animal models and humans.67
Beginning with an angiogenic stimulus, the angiogenic process involves a number of discrete yet overlying steps (Fig. 79-7). Initially, the vessels become dilated and permeable with retraction of the endothelial cells and a decrease in endothelial junctions. The basement membrane is then dissolved by proteases, and the endothelial cells migrate from the vascular wall toward the angiogenic stimulus. Behind the leading front of migrating endothelial cells, endothelial cell replication begins, forming a capillary sprout that elongates toward the angiogenic source. The nearby capillary sprouts then anastomose to each other, forming capillary loops. As capillary loops and sprouts continue, the loops become patent, developing newly formed blood vessels. These blood vessels differentiate and lay down basement membrane consisting of type IV collagen, laminin, and proteoglycans. Pericytes and fibroblasts then migrate to the capillary loop sites.68
With the continued presence or absence of the angiogenic stimulus, substantial remodeling, regression, and rearrangement of the new capillaries occur.69 Some capillaries join preexisting flap vessels (inosculation), but the majority of revascularization appears to involve direct ingrowth of recipient vessels into the flap (Fig. 79-8). New capillaries can grow toward an angiogenic source at a mean rate of 0.2 mm/day. When the angiogenic stimulus is discontinued, the capillary vessels regress and eventually disappear over a period of weeks. Angiogenic growth factors can stimulate capillary growth over distances of 2 to 5 mm.70 It is believed that mechanisms to inhibit angiogenesis exist to prevent an uncontrollable cascade of neovascularization. Evidence suggests that pericytes can suppress endothelial growth by direct contact.66 Thus, the physiologic response of angiogenesis may be analogous to the blood coagulation pathway that should be maintained at a steady state of control. In soft-tissue wound repair, macrophages, lymphocytes, mast cells, and platelets are involved in releasing various factors that modulate angiogenesis.71,72
Nerve Section
Sensory and sympathetic nerves are severed in the process of flap elevation. Although loss of sensation may limit the usefulness of the flap after transfer, adrenergic denervation has implications for flap survival. When a sympathetic nerve is divided, catecholamines are released from the nerve terminal, and the mechanism for catecholamine reuptake is eliminated.73–75 A local “hyperadrenergic state” exists, which produces vasoconstriction mediated by α-adrenergic receptors in the cutaneous vasculature.73
The vasoconstricting effect of sympathectomy further reduces total flap blood flow.76,77 which has already been decreased by division of supplying vessels. This further reduction negatively affects the ratio of perfusion pressure to the critical closing pressure of the arterioles in the subdermal plexus. A greater proportion of the distal flap is excluded from the blood supply, and necrosis becomes more likely. The stored transmitter is depleted within 24 to 48 hours,73 and blood flow increases as the concentration of norepinephrine declines.77 In critical areas of the flap, the time to recovery of nutrient blood flow may be delayed sufficiently to produce additional necrosis.
Inflammation and Prostaglandins
The surgical trauma and ischemia associated with an acutely raised flap result in an inflammatory response. Histamine, serotonin, and kinins are released into the extracellular compartment after flap elevation, increasing the permeability of the microcirculation. The result is a rise in the concentration of proteins and cells within the extracellular space. The presence of nonbacterial inflammation beginning a few days before flap elevation has been shown to improve flap survival,78–80 presumably as a result of an increase in local blood flow.
The inflammation created during flap elevation may have deleterious effects because of the resultant edema formation. In addition to compromising capillary blood flow and altering the relationships of the Starling equation, interstitial edema increases the diffusion distances between cells. The effect is enhanced because of the inverse square law for diffusion. Edema also can constitute a direct barrier to diffusion.23,29
Prostaglandins are derived from essential fatty acids that are incorporated in membrane phospholipids (Fig. 79-9). Activation of phospholipases results in the production of prostaglandin (PG) H2 by cyclooxygenase. Prostaglandins E1 and E2 can be synthesized from PGH2 by isomerases in the vascular endothelium. PGE1 and PGE2 produce vasodilation. PGD2 also is formed by an isomerase reaction and is the principal cyclooxygenase product of the mast cell. Its effects on the cutaneous microvasculature are similar to those of PGE1. Prostacyclin (PGI2) is a vasodilating agent and inhibitor of platelet aggregation that is derived from PGH2 through the action of prostacyclin synthase. In the skin, PGI2 is produced primarily in the endothelial cells of blood vessels.81,82
Thromboxane synthetase, which converts PGH2 into thromboxane (Tx) A2, is located primarily in the platelets. Its effects include vessel constriction and promotion of platelet aggregation.83 TxA2 is unstable and is rapidly converted into TxB2. PGF2α is derived from PGH2 by a reductase reaction. A marked increase in resistance is seen in cutaneous arteries, arterioles, and venules in the presence of PGF2α.84 The synthesis of prostaglandins and thromboxane can be altered by pharmacologic manipulation. The action of phospholipase A2 can be inhibited by drugs that reduce the availability of calcium. Glucocorticoids also affect phospholipase A2 activity by inducing the synthesis of a protein that inhibits the enzyme.85 Aspirin and other nonsteroidal anti-inflammatory drugs (NSAIDs) interfere with the cyclooxygenase enzyme, inhibiting the synthesis of PGH2.
Prostaglandins clearly play a role in the inflammatory response after flap surgery. Prostacyclin levels increase after flap elevation, with a peak concentration around 7 days, and then decrease to postoperative day 21.81 PGE2, PGF2α, and TxB2 levels also rise after the creation of a flap.86 The increase in PGE2, PGF2α, and TxB2 can be blunted by creation of a bipedicled flap. Conversion to a single-pedicle flap (“delay”) results in decreased levels of thromboxane and a higher level of PGE2, which remain elevated for at least 7 days.87 Whether these changes in prostaglandin levels represent a cause or a side effect of the observed phenomenon remains to be seen.
Reperfusion Injury
Controversy and, often, confusion surround the terms no-reflow and reperfusion injury. No-reflow phenomenon connotes the condition in which zone I perfusion has been reestablished but zone II or III failure prevails.76,80,83,86,88–90 Reperfusion injury describes the observation that tissues tolerate short periods of total ischemia fairly well but exhibit histologic injury after return of perfusion—thus, injury apparently caused by reperfusion.91,92 Both terms imply a period of ischemia, and both conditions result in microcirculatory failure.
All flaps experience some ischemia, and return of blood flow may result in microcirculatory impairment.53,93 Free radicals form during reperfusion and cause tissue injury, but other factors, such as hyperosmosis from lactic acid accumulation,94 have also been implicated.95,96
Free Radical Formation
When oxygen becomes available with reperfusion, an additional menace to flap survival is produced—the free radical. This byproduct of reperfusion can cause damage at the cellular and subcellular levels,97,98 contributing to postischemic tissue necrosis. The neutrophil appears to play a major role in the mediation of reperfusion injury.99
Free radicals are extremely reactive compounds because of an unpaired electron in their outer orbits. Oxygen free radicals are formed by the sequential univalent reduction of molecular oxygen. The superoxide anion radical (O2−) is formed by the addition of a single electron to molecular oxygen. Superoxide is a byproduct of ATP production in the mitochondria and other oxidation-reduction reactions.98 Polymorphonuclear cells, a second source of superoxide radicals, are released in response to bacterial inflammation.97
A major source of free radicals in ischemic tissue is the enzyme xanthine oxidase (Fig. 79-10).100 With ischemia, high-energy phosphate compounds are converted to hypoxanthine, which accumulates in the tissues. When oxygen becomes available with reperfusion, xanthine oxidase catalyzes the conversion of hypoxanthine into uric acid, producing superoxide in the process. This reaction is believed to be an important mechanism in ischemic tissue injury in skin flaps.50 The role of xanthine oxidase has been brought into question by the fact that some investigators have found xanthine oxidase activity in ischemic skin27,101 but others have not.102 Xanthine oxidase activity has been found in normal rat skin and increases its activity after venous occlusion, reperfusion,27 and flap elevation, with the highest levels present distally.43 Tissue damage resulting from production of free radicals can occur through lipid peroxidation of the cellular membrane and denaturation of the intracellular matrix.98 Delayed neovascularization has been proposed as another consequence of free radical damage affecting proliferating endothelial cells.103
Capillary Obstruction (No-Reflow Phenomenon)
Arterial and random flaps can tolerate several hours of total avascularity and remain viable.104 When the critical ischemia time for a flap is exceeded, an ischemia-related obstruction to blood flow, known as the no-reflow phenomenon, develops. Even though large vessels (zone I) have adequate flow, there is no perfusion in zone II or III. The no-reflow phenomenon was described in skin flaps by May and colleagues.105 Swelling of the endothelial and parenchymal cells, coupled with intravascular stasis and eventual thrombosis, leads to loss of nutritive flow. Interactions between polymorphonuclear cells and endothelial cells appear to play a fundamental role in the generation of a reperfusion injury.96 Both cell groups produce cytokine and proadhesive molecules that affect the inflammatory response.93 Polymorphonuclear cells adhere to the vascular wall and generate proteases and oxygen free radicals that injure the endothelial cells.106
Flap Biomechanics
Skin flap biomechanics are governed principally by collagen and elastin.107,108 The interplay of these components has a direct influence on the results of flap wound closure. Collagens I and II constitute the principal structural framework of the extracellular matrix.109 Elastic fibers, meanwhile, are responsible primarily for the elasticity and compliance of the skin. When skin is stretched, the fine elastin network initially allows easy deformation. This is demonstrated on the stress-strain curve (Fig. 79-11).110 As the force (stress) applied to the skin increases, randomly arranged collagen fibers begin to lengthen in the direction of the force and deformation becomes more difficult (section II). Further lengthening becomes difficult. Once the collagen fibers have all become oriented longitudinally with the force, little further deformation is possible with increasing stress (section III). Skin in section III of the curve behaves like elderly skin, suggestive of the maintenance of collagen biomechanics with aging. As patients age, there is a progressive decrease in elastic fibers.111 Thus, less force is necessary to produce lengthening. Older patients can therefore be expected to have less wound tension.
Wound tension is an important factor in flap survival as well as scar formation. Excessive tension in skin flaps is associated with greater flap necrosis.112,113 This is probably secondary to a drop in blood flow with increasing wound tension.
Creep refers to the increase in strain (change in length relative to original length) seen when a constant stress (force) is applied to the skin. Stress relaxation, on the other hand, refers to the decrease in stress that occurs when skin is held under tension at a constant strain.3,114 Thus, the physiology of skin is altered over time by the application of a constant stress. These properties are responsible for the effects seen with tissue expansion and serial excisions of lesions.
Flap undermining is routinely performed during flap elevation and wound closure. It is the release of the attachments of the skin to the underlying tissue that results in a decreased wound tension when undermining is performed.115 The force required to counteract the resistance between the dermis and underlying tissue is the shearing force. In an animal model, the reduction in the shearing force, and thus wound tension, was found to decrease with undermining up to 4 cm. Further undermining did not produce more improvement in wound tension.114
Attempts to Alter Skin Flap Viability
Increased Blood Supply
Skin flap failure can occur from extrinsic and intrinsic causes.116 Extrinsic reasons for flap necrosis are reasons not resulting from the design of the raised flap. Examples are systemic hypotension, infection, and pedicle compression. These factors often can be overcome in the clinical situation. The primary intrinsic factor affecting flap survival is inadequate blood flow. Numerous experimental attempts have been made to influence flap microcirculation or decrease the deleterious effects of inadequate flap blood flow (Fig. 79-12). The most successful has been flap delay. Table 79-1 shows the zones of perfusion affected by various interventions to increase flap survival.
Table 79-1 Zones of Perfusion Affected by Various Interventions to Increase Flap Survival
Intervention | Affected Zone(s) |
---|---|
Flap design | I |
Delay | I, II |
Vasodilators | II |
Neovascularization | II |
Rheology | II |
Inflammation | II, III |
Antioxidants | II, III, IV |
Metabolic manipulation | II, IV |
Increased oxygenation | IV |
Delay
Four things are accepted about the delay phenomenon. First, surgical trauma is required to cause it. Second, a large percentage of the neurovascular supply to the flap should be eliminated. Third, delay results in increased flap survival at the time of tissue transfer. Fourth, the beneficial effects can last up to 6 weeks in humans.117 To explain this phenomenon, three theories regarding the mechanism of delay have been developed: (1) delay improves the blood flow, (2) delay conditions the tissue to ischemia, and (3) delay closes arteriovenous shunts.118 Most later studies support a mechanism resulting in increased circulation to the flap; how this occurs remains to be proven.117
The percentage of arteriovenous shunt flow to total blood flow is similar in delayed flaps and acute flaps.77 Delayed flaps simply have an increase in total blood flow. The addition of systemic norepinephrine decreases the blood flow in delayed flaps to the level seen in acute flaps. Thus, one effect of delay is a decrease in vasoconstriction in the distal portion of the flap.
Flaps delayed as few as 24 hours survive to a greater length and can tolerate longer periods of ischemia.119,120 Distal perfusion in the pig flank flap increases with a bipedicled flap delay up to 4 days. No further increase in perfusion occurs, extending delay up to 14 days.121,122 Pang and colleagues77,93 theorized that after a delay, reduced vasoactivity of the small arteries allows delivery of more blood to the distal portion of the flap. This modulation could occur by release of vasoconstrictive substances (i.e., norepinephrine, thromboxane, and serotonin) during elevation of the bipedicled flap. Degeneration release of norepinephrine occurs soon after flap elevation, with norepinephrine stores largely depleted in the first 24 to 48 hours.82,123 In bipedicled flaps, Cutting and associates121,124
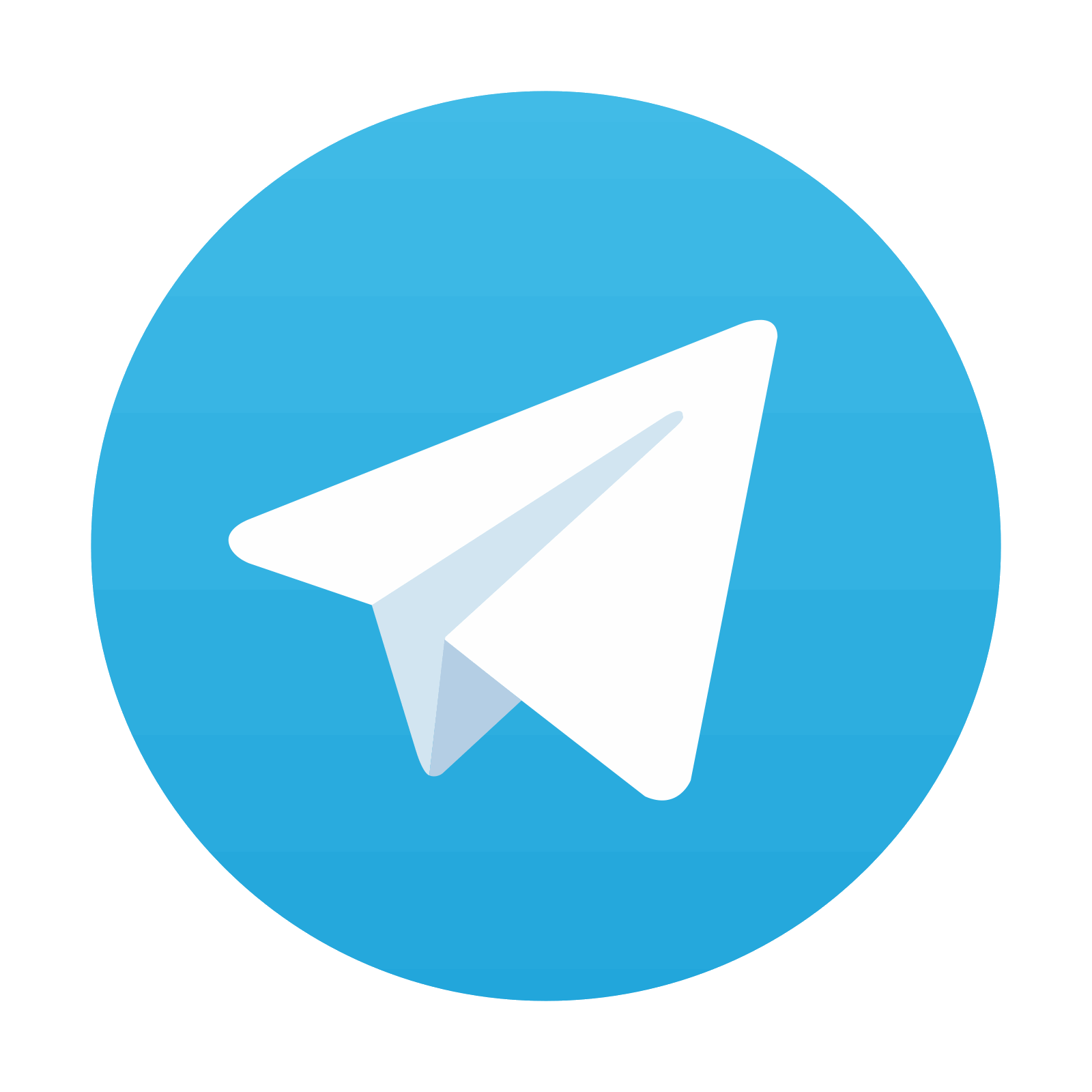
Stay updated, free articles. Join our Telegram channel
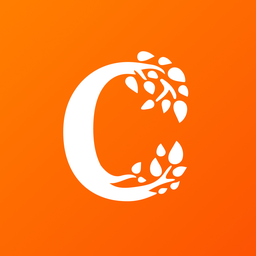
Full access? Get Clinical Tree
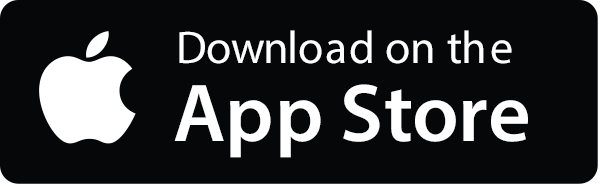
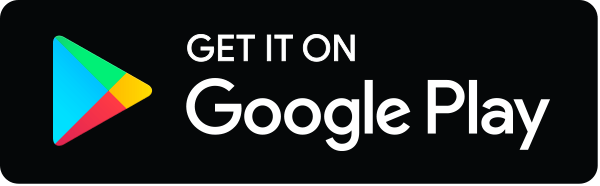