CHAPTER 84 Physiology of the Salivary Glands
Throughout the history of science, the salivary glands have been a topic of keen biologic interest and questions regarding their mechanisms of function have spurred numerous research endeavors. As early as 160 AD, Galen described the position of the major and minor salivary glands and their respective ductal openings. In 1543 Vesalius published a more detailed anatomic description of the salivary glands in a monograph entitled De Humani Corporis Fabrica. Interestingly, however, before the 17th century the salivary glands were thought to serve only as emunctories, the function of which was to strain excrementitious substances such as the evil spirits of the brain from the blood. Fortunately, the fortitude and dedication of scientists such as Boredu, Ludwig, Langley, Haller, Heidenhain, Mueller, Baylis, Bernard, and Pavlov allowed this notion to be dispelled and the field of salivary gland physiology to be advanced.1 Recent technologic advances, along with a new generation of scientists, have brought this field even further, expanding our knowledge of the mechanisms of salivary gland function, disease processes that impede normal gland function, and methods by which to restore homeostasis in a diseased gland. Importantly, our current understanding of salivary gland physiology is largely derived from the study of nonhuman salivary glands.
Saliva is a complex mixture of electrolytes and macromolecules secreted from three pairs of major and numerous minor salivary glands. The major salivary glands consist of the parotid, submandibular, and sublingual glands. The minor salivary glands line the mucosa of the lip, tongue, palate, and pharynx. The secretion of saliva is tightly modulated by a multitude of factors including the autonomic nervous system, humoral factors, and disease states. Saliva performs a number of diverse and crucial roles: (1) It provides lubrication that aids in swallowing; (2) it is an emulgent that aids in the digestion of food and enzymatic cleavage; (3) it produces hormones, hormone-like substances, and other metabolically active compounds; (4) it assists excretion of endogenous and exogenous materials such as antibodies, blood-group reactive substances, iodine, and viruses; (5) it mediates taste sensations; and (6) it provides defense against bacterial pathogens. Relative to size, the salivary glands produce a large volume of saliva. The maximal rate of saliva production in humans is about 1 mL/min/g of glandular tissue. The rate of metabolism and blood flow to the salivary glands is also high, proportional to the rate of saliva secreted. To put this in perspective, the flow of blood to a maximally secreting salivary gland is approximately 10 times greater than the flow of blood to an equal mass of actively contracting skeletal muscle.2 This chapter provides a detailed discussion of the various aspects of salivary gland function and physiology.
Principles of Salivary Gland Secretion
Anatomy of the Secretory Unit
The basic unit of the salivary gland consists of an acinus, a secretory duct, and a collecting duct (Fig. 84-1A and B). In general the area of the acinus and proximal secretory duct is called the secretory endpiece. The secretory duct is composed of the intercalated and striated ducts, which are intralobular. The excretory and collecting ducts are extralobular. The structural relationships and secretory capabilities of secretory units within different salivary glands differ widely. The parotid and submandibular glands have a single, elongated, large-caliber collecting duct, with only a few major branching interlobular ducts. These interlobular ducts in turn are connected to many intralobular ducts, each of which transports saliva from several acini. In contrast to the parotid and submandibular glands, the secretions of the sublingual gland are discharged through 10 to 12 separate collecting ducts. The minor salivary glands, which are essentially groups of individual secretory units, are distributed throughout the submucosa of the oral cavity and have short convoluted collecting ducts.2
The acinus comprises a central lumen surrounded by pyramidal-shaped cells (see Fig. 84-1A and B). These acinar cells are highly polarized and are bounded by a plasma membrane with two distinct domains, a basolateral domain and an apical domain. These two domains are functionally and physically separated by tight junctions that link adjacent cells just below the luminal area. Each acinus is surrounded by a layer of myoepithelial cells, which in turn is delimited by a distinct basement membrane layer. Myoepithelial cells are elongated or star-shaped nonsecreting cells with long-branching processes that surround the acinus and proximal ducts. The observation that myoepithelial cells possess adenosine triphosphate activity, have intercellular gap junctions, and contain myofilaments has led to the hypothesis that these cells have contractile properties and play a role in expelling preformed secretions.3–5
Acini are classified as serous, mucous, or mixed (Table 84-1). Serous acini contain pyramidal-shaped cells with round basal nuclei surrounding the lumen. The cytoplasm of the serous cells is densely packed with basophilic secretory granules, poised to discharge their contents into the acinar lumen. The number of these granules varies with the phase of secretory activity, decreasing after a period of secretion and reaccumulating following a period of synthesis. Mucous acini have a larger lumen than serous acini, and the cells surrounding the lumen have a clear cytoplasm, flattened basal nuclei, and numerous droplets of mucigen, which is the precursor of mucin. In mixed salivary glands, acini are composed of both serous and mucinous cells. Mucinous cells are found surrounding the lumen of the acini, whereas serous cells are found near the fundus of the acini forming a caplike structure, called a serous demilune.6
The acinus is contiguous with the intercalated duct, a hollow structure lined by a single layer of small cuboidal cells (see Fig. 84-1A and B). A layer of myoepithelial cells surrounds these cuboidal cell–lined ducts, similar to acini. The intercalated ducts continue as striated ducts, which are lined by columnar cells and have a brush border composed of microvilli on their luminal surface. Striated ductal cells also have prominent basal striations formed by infoldings of the plasma membrane that enclose columns of rod-shaped mitochondria. The high-energy characteristics of these cells suggest that they are involved with the transport of ions and water. The striated ducts lead into excretory ducts, which are lined by two layers of epithelium, a layer of flat cells surrounding the ductal lumen, and an outer layer of columnar cells.6,7
Secretory Process
Saliva consists of a complex mixture of electrolytes and macromolecules. It is now known that saliva is formed via active transport processes occurring throughout the secretory unit and that these processes are under the control of complex neuronal and hormonal signals. The secretory unit consists of two anatomically and functionally distinct regions: the acinus and the secretory duct. The acinus is the site of all fluid generation and most (≈85%) exocrine protein secretion.7 The fluid component is derived from the local vascular bed in the form of an isotonic solution and secreted into the acinar lumen. This primary secretion traverses the ductal system before emptying into the mouth. In contrast to the water-permeable cells of the acinus, ductal cells are water impermeable. Most of the sodium (Na+) and chloride (Cl−) in the primary secretion is reabsorbed in the duct, and a small amount of potassium (K+) and bicarbonate (HCO3−) is secreted. In addition, some proteins are added to the salivary fluid as it traverses the secretory duct. By the time the saliva enters the mouth, it has generally been rendered hypotonic (≈25 mEq/L NaCl). The electrolyte composition of saliva, however, can be influenced by salivary flow rates. The reabsorption of salivary sodium and chloride is directly related to these rates, with decreased reabsorption and increased salivary concentrations of electrolytes with increasing salivary flow rates. Potassium reabsorption is independent of flow rates.8,9
Mechanisms of Primary Fluid Secretion
Fluid transport in salivary glands is thought to be driven osmotically in response to transepithelial salt gradients. These salt gradients are generated by ion transport systems localized to the luminal and basolateral membranes of the acinar cell. On the basis of studies in rabbit and rat salivary glands, three mechanisms for primary fluid secretion by the acini have been proposed. These mechanisms (Fig. 84-2) appear to operate concurrently in the same gland, and possibly in the same acinar cell. The relative importance of each of these mechanisms varies from species to species, gland type to gland type, and possibly from physiologic status to physiologic status.
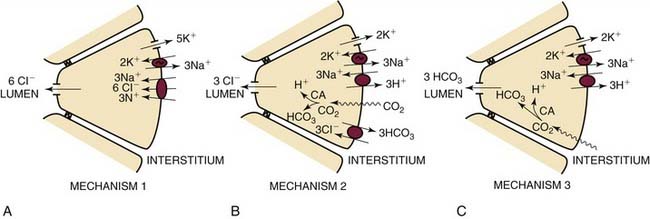
Figure 84-2. Schematic representation of three mechanisms of primary fluid secretion in acinar cells.
(From Turner RJ. Mechanisms of fluid secretion by salivary glands. Ann N Y Acad Sci. 1993;694:24.)
Fluid secretion in the acini is the result of the combined action of four membrane transport systems: (1) a Na+ − K+ − 2Cl− cotransporter located in the basolateral membrane of the acinar cell; (2) a calcium (Ca++)-activated K+ channel in the basolateral membrane; (3) a calcium-activated Cl− channel localized to the apical membrane; and (4) an adenosine triphosphatase (ATPase)-driven Na+/K+ pump in the basolateral membrane. In the resting or unstimulated state, both K+ and Cl− are concentrated in the acinar cell above the electrochemical equilibrium, the former by the Na+/K+ ATPase and the latter via the Na+ − K+ − 2Cl− cotransporter.9,10
In the first mechanism of saliva secretion (see Fig. 84-2A), stimulation by the autonomic nervous system leads to a rise in intracellular calcium concentration, which results in the opening of the basolateral Ca++-activated K+ channels and the apical calcium-activated Cl− channels. This increase in K+ and Cl− conductance allows KCl to flow out of the cell, resulting in an accumulation of Cl− ions and their associated negative electrical charge in the acinar lumen. Sodium in the interstitium then follows Cl−, due to electrical attraction, by leaking through the tight junctions between the cells into the acinar lumen. The resulting osmotic gradient for NaCl causes a transepithelial movement of water from the interstitium into the acinar lumen. In the continued presence of the agonist, a net transepithelial chloride flux and a concomitant secretion of fluid are sustained due to Cl− entry via the Na+ − K+ − 2Cl− cotransporter and exit via the apical Cl− channel. When the stimulus is removed, the intracellular calcium concentrations fall to resting levels, the K+ and Cl− channels close, and the cell returns to its resting state. In order for this model to operate continuously, it must satisfy the constraints of mass and charge balance. In other words, in the steady state ion transport must be such that neither mass nor charge is continually accumulated in or depleted from the cell. These constraints, along with the known stoichiometries of the Na+ − K+ − 2Cl− cotransporter (1Na+:K+:2Cl−) and the Na+/K+ ATPase (3Na+:2K+:1ATP), determine the relative fluxes of ions per cycle: Six chloride ions are translocated from the interstitium to the acinar lumen per ATP molecule hydrolyzed by the Na+/K+ ATPase.9
Mechanism 2 (see Fig. 84-2B) is similar to mechanism 1, except that in this model a basolateral Cl−/HCO3− exchanger, in parallel with an Na+/H+ exchanger, replaces Na+ − K+ − 2Cl− cotransporter. Decreases in intracellular chloride concentrations resulting from secretagogue-induced KCl loss lead to increased Cl− entry in exchange for HCO3− via the Cl−/HCO3− exchanger. The cytoplasmic acidification resulting from this bicarbonate loss is buffered by the Na+/H+ exchanger, which uses the extracellular-to-intracellular sodium gradient generated by the Na+/K+ ATPase to drive protons out of the cell. The net result is the movement of NaCl into the cell in exchange for H2CO3, which in turn is recycled across the basolateral membrane as CO2 after hydrolysis by carbonic anhydrase. In this model, three chloride ions are translocated from the interstitium to the acinar lumen per ATP molecule hydrolyzed. Sodium and water follow the chloride into the acinar lumen from the interstitium.9
In contrast to mechanisms 1 and 2, in which chloride is the secreted anion, mechanism 3 (see Fig. 84-2C) involves apical bicarbonate secretion. In this model, carbon dioxide (CO2) enters the acinar cell across the basolateral membrane and is converted to HCO3 plus a proton by carbonic anhydrase. The bicarbonate is lost across the apical membrane via an anion channel, possibly the same channel involved in chloride secretion, and the protein is extruded by the basolateral Na+/H+ exchanger. In this model, three bicarbonate ions are secreted per ATP molecular hydrolyzed. Sodium and water follow the secreted bicarbonate ions into the acinar lumen.9
Mechanisms of Primary Macromolecule Secretion
The protein subcomponent of saliva is derived primarily from secretory granules of the acinar and ductal cells. Secretory proteins are discharged into the lumen of the secretory unit by a process of exocytosis, wherein fusion of secretory granules with a delimited portion of the plasmalemma of the apical membrane occurs. The membrane fusion is the last of a series of steps required for the transfer of export proteins from their synthesis in the rough endoplasmic reticulum (RER) to the extracellular environment. According to the model by Palade, the secretary process can be divided into six steps: (1) synthesis, (2) segregation, (3) intracellular transport, (4) concentration, (5) intracellular storage, and (6) discharge.11
The synthesis of secretory proteins requires the uptake of amino acids by the cell, which is accomplished via an active transport mechanism in the basolateral surface of the cell. Transfer ribonucleic acids (tRNA) then deliver the amino acids to the ribosomes of the RER, where messenger ribonucleic acids (mRNA) are translated into polypeptides. Before transport of these polypeptides from the RER to the Golgi apparatus, they undergo some post-translational modifications and are segregated in the cisternal space of the RER. Segregation is regarded as an irreversible step. From the RER, polypeptides are transported to the Golgi apparatus via an ATP-dependent mechanism. In this apparatus, polypeptides undergo further post-translational modifications such as terminal glycosylation and are concentrated. At this step the polypeptides are transferred from a high-permeability membrane to a membrane with characteristics similar to the plasmalemma, forming secretory granules. On appropriate stimulation from the autonomic nervous system (discussed later), the secretory granules discharge their contents into the glandular lumen by the process of exocytosis. The membrane of the secretory granule fuses with the plasmalemma, bringing into continuity the contents of the secretory granule and the extracellular lumen, and at the same time maintaining a diffusion barrier between the interior of the cell and extracellular medium. The mechanism by which the cell recycles the apical membrane is not completely understood but likely involves the process of endocytosis and the selective degradation of endocytic vesicles.12
Mechanisms of Ductal Secretion
Ductal secretion is not constant and the underlying mechanisms are only partially understood. Microperfusion studies of excretory ducts have confirmed the ability of ductal cells to modify saliva by reabsorbing sodium and chloride and secreting potassium and bicarbonate to produce the final hypotonic solution.13 In addition, ductal cells have the ability to secrete some protein into the ductal lumen. In general, when the salivary flow rate is slow, more time is available in which ion transfer can occur across the tubular cells, resulting in greater modification of the secretory fluid. When the flow rate is high, however, the contact period is shortened, diminishing the influence of the tubular cells on solute concentration. The exception to this rule is that conditions that stimulate increased flow rates also stimulate increased bicarbonate secretion.2,6
Neuronal Control of Secretion
In general, significant secretion from the human salivary glands occurs only in response to stimulation by the autonomic nervous system or by the action of substances that can mimic the effects of such stimulation.14 Both sympathetic and parasympathetic nerves innervate the salivary glands, though the effects of the parasympathetic nerves predominate. Nevertheless, it is likely that the two components of the autonomic nervous system have a synergistic effect on salivary gland function. Parasympathetic stimulation is the principal impetus for salivary gland fluid secretion. In addition, parasympathetic stimulation leads to some exocytosis and protein secretion, myoepithelial contraction, and vasodilation. Sympathetic stimulation is a weak mobilizer of salivary fluid, though its effect may be additive to that of the parasympathetic system. On the other hand, sympathetic stimulation causes high levels of protein secretion, myoepithelial contraction, and maintenance of vascular tone. In general, parasympathetic stimulation leads to the output of saliva that has a large volume and low protein content, whereas sympathetic stimulation leads to the secretion of low volumes of saliva with high protein content.2,6,12
The innervation patterns of the major salivary glands differ considerably, from species to species, subject to subject, and cell type to cell type. The parasympathetic supply to the parotid gland originates in the inferior salivatory nucleus and travels with the glossopharyngeal nerve, and then with Jacobson’s nerve to the otic ganglion, where it synapses. The postganglionic fibers are carried by the auriculotemporal branch of the trigeminal nerve to the parotid gland. The parasympathetic supply to the submandibular gland originates in the superior salivatory nucleus and travels via the nervus intermedius and chorda tympani to the submandibular ganglion. Some of these fibers synapse in the ganglion, whereas others synapse in the gland itself. The sympathetic supply to the parotid and submandibular gland originates from the superior cervical ganglion and follows major arterial blood vessels to reach the various salivary glands. Nerve fibers of the parasympathetic and sympathetic nervous system are distributed in a similar fashion around the acini, intercalated ducts, and striated, though the parasympathetic fibers predominate.15 Myoepithelial cells are similarly liberally innervated, whereas the collecting ducts are sparsely innervated. In contrast to other organ systems such as skeletal muscles, which contain a well-defined neuronal synapse at the axon-effector organ interface, secretory cells have unmyelinated fibers of the parasympathetic and sympathetic system that lie in close proximity to the effector cell. These axonal fibers can lie either outside (epilemmal fibers) or inside the basement membrane (hypolemmal fibers) of the effector cell.16 Neurotransmitters released from the axon presumably reach the secretory cell by diffusion. These neurotransmitters, as well as other hormones and regulatory molecules, affect the function of salivary cells in a complex manner that is poorly understood.
Neurotransmitters and Receptors
Cell surface receptors are macromolecular moieties within the cell membrane that bind ligands such as neurotransmitters in the extracellular milieu. Ligand binding activates the receptor, which then transmits a signal across the cell membrane and triggers a biologic response within the target cell (Fig. 84-3). The biologic response can be initiated directly by the receptor or, more commonly, mediated through a second messenger system activated by the receptor-ligand complex. The specific biologic response initiated and the magnitude of the response is a function of the receptor itself and not the ligand with which it binds. Receptors for neurotransmitters are typically located on the basal and lateral surfaces of the secretory cells of the salivary gland.
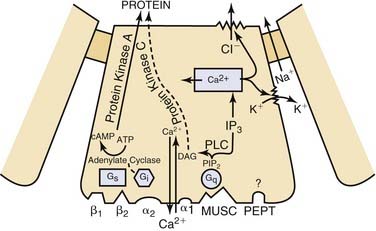
Figure 84-3. Schematic representation of acinar cell receptors and second messenger systems.
(From Baum BJ. Principles of saliva secretion. Ann N Y Acad Sci. 1993;694:17.)
The neurotransmitter acetylcholine mediates the effects of the parasympathetic nervous system, whereas norepinephrine mediates the effects of the sympathetic nervous system. Adrenergic receptors, receptors for norepinephrine, are divided into two major classes, alpha and beta. Each alpha and beta class is further divided into two subtypes, yielding α1 and α2 and β1 and β2 receptors (Table 84-2).17 The best-studied acinar cells, those of the rat parotid gland, appear to have all four subtypes of adrenergic receptors, though in general the functionally important receptors appear to be the α1 and β1 subtypes. The β1 and β2 receptors are linked to the adenylate cyclase second messenger system, and binding of ligand to this receptor activates adenylate cyclase. Alpha-2 receptors are also linked to the adenylate cyclase system; however, binding of ligand to this receptor leads to the inhibition of adenylate cyclase. Alpha-1 receptors are linked to a yet uncharacterized second messenger system that does not regulate adenylate cyclase but instead modulates calcium influx.7
Cholinergic receptors, receptors for acetylcholine, are divided into two categories: muscarinic and nicotinic. Acinar cells contain only muscarinic receptors, specifically the muscarinic receptor M3 subtype. Binding of acetylcholine to the M3 receptor leads to activation of the phospholipase C pathway and ultimately intracellular calcium mobilization. Recent evidence suggests the presence of two additional subtypes of muscarinic receptors in the rat submandibular and sublingual gland, M1 and M5.18 The effect of these two receptors on acinar cell function and the mechanism by which these effects are mediated are unclear at this point. Some of the effects of the M1 receptor on salivary gland function may be indirect via nitric oxide.7
Parasympathetic-mediated nonadrenergic, noncholinergic secretory response and vasodilation are well-known phenomena in salivary glands, and a number of polypeptides including neuropeptide Y (NPY), vasoactive intestinal peptide (VIP), galanin (GAL), substance P (SP), and calcitonin gene-related peptide (CGRP) have been implicated in mediating this pathway.19 NPY-, VIP-, and GAL-immunoreactive nerve fibers are densely distributed around acini and ducts. CGRP- and SP-immunoreactive fibers are also found around these structures, but to a lesser extent. Interestingly, the density of SP- and CGRP-immunoreactive fibers around the mucous acini was significantly higher than around serous acini. The VIP system is the best studied of these polypeptide neurotransmitters in salivary glands and is similar to what has been described in the gut and pancreas.8 Currently, the role of these different polypeptide neurotransmitters in salivary gland function is speculative; however, in view of their potential therapeutic roles, intense efforts are under way to better understand them.19
Signal Transduction
The formation of a receptor-ligand complex is only the first step toward initiating a biologic response within the target cell. The second step entails transmission of the ligand-receptor binding signal across the cell membrane and activation of a second messenger system. The second messenger system then activates an effector system, bringing about a specific biologic response (see Fig. 84-3).
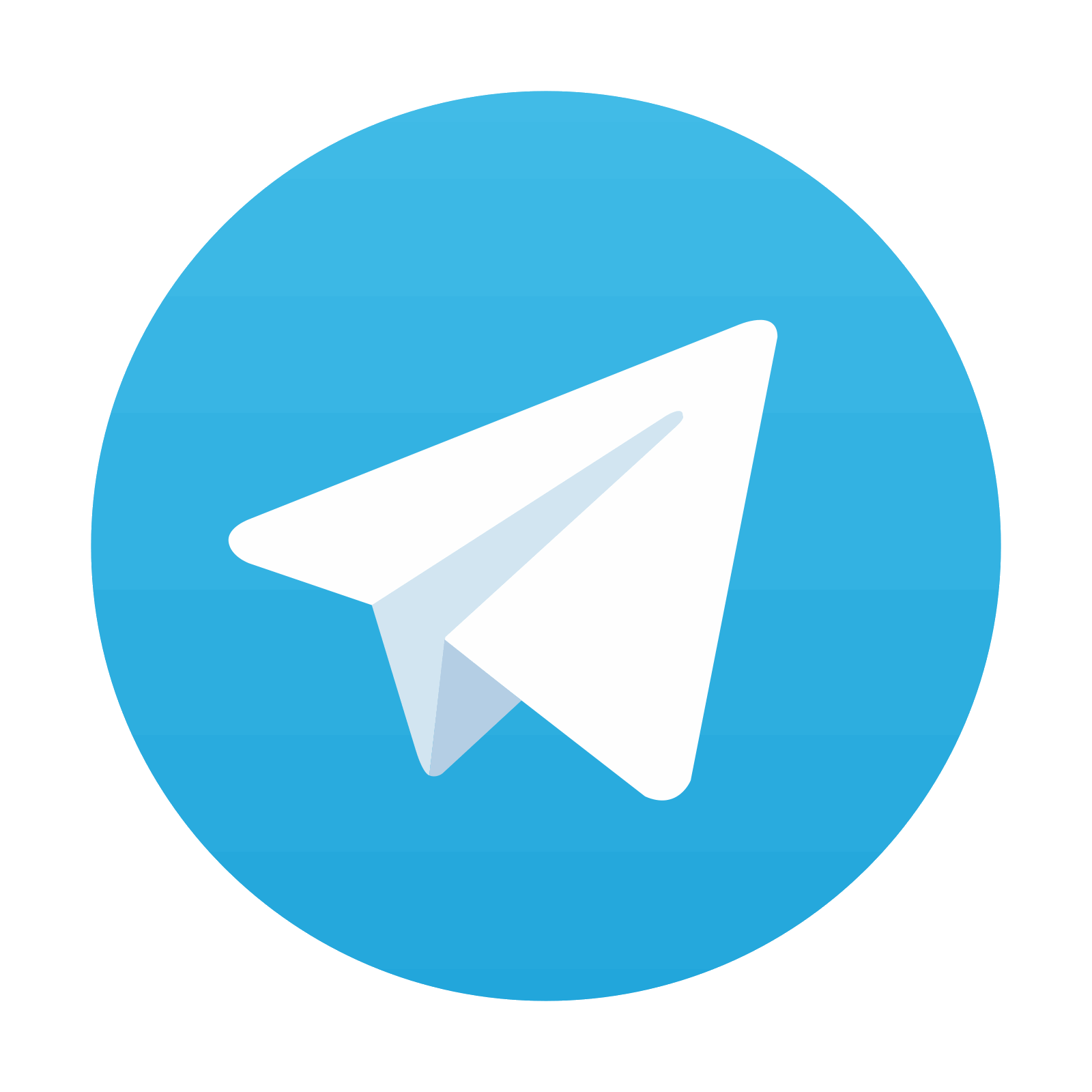
Stay updated, free articles. Join our Telegram channel
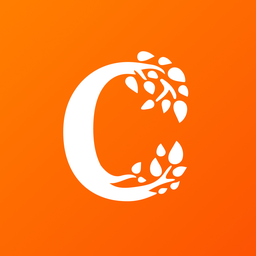
Full access? Get Clinical Tree
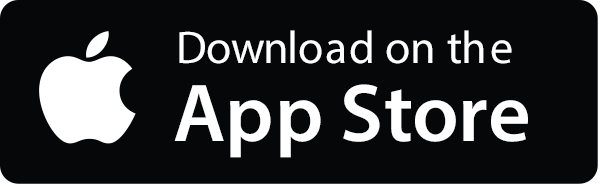
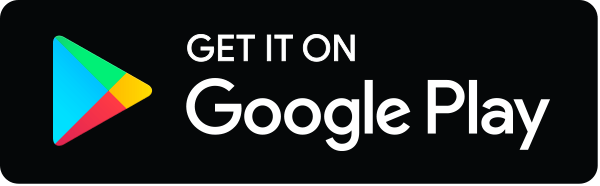