CHAPTER 89 Physiology of the Oral Cavity
The oral cavity is a complex organ comprising muscle, glands, teeth, and specialized sensory receptors. For most animals, the orosensory and oromotor apparatus is critical for successful defense, reproduction, exploration, nutrition, and vocalization.1 In humans, vocalization has evolved into complex speech production, but other human behaviors depend less on the mouth and tongue than on the eye and hand. In all animals, however, the mouth is essential for the ingestion of nutrients. The incorporation of nutrients by mastication and drinking involves a high degree of coordination within and between different oral motor systems. Chewing requires the reciprocal activation of antagonist trigeminal muscles to open and close the jaws and the tongue to position food between the teeth. A diverse array of highly specialized sensory systems guides these complex oromotor responses and initiates secretion of digestive enzymes. Mechanoreceptors in the tongue, palate, and periodontal ligament (PDL) all contribute to a three-dimensional (stereognostic) perception of the oral cavity.2 The sense of taste serves in both food selection and protection from ingesting potentially toxic substances.
Recent reviews provide comprehensive coverage of specific aspects of oral function including mastication,* swallowing,3,4 oral mechanoreception,5,6 and the sense of taste.7–10 In addition, several recent papers have reviewed oral pain11,12 and taste dysfunction.13
Sensory Function
Oral Somesthesia
Overall, the anterior oral cavity displays greater tactile sensitivity than does the posterior oral structure.1,14 The tip of the tongue is particularly sensitive, with a discriminative capability equivalent to that of the digits (Fig. 89-1). The midline of the palate and tongue are more sensitive than lateral regions. A similar pattern of sensitivity applies to the teeth.15 Adults with complete dentition could detect a 1-g von Frey hair applied to the anterior (midline) teeth but require nearly 10 g to detect stimulation of the first molar. The sensitivity to warm and cold stimuli also varies widely across oral tissues. Sensitivity to warm stimuli is relatively high on the tip of tongue but not particularly so on either the palate or buccolabial surfaces.16,17 In contrast, the sensitivity to a cool stimulus is less differentiated within the oral cavity, and the sensitivity of the tongue tip, palate, and buccolabial surfaces is essentially equal. In general, the sensitivity to cool stimuli is greater compared with warm stimuli.
Recording from single human lingual fibers innervating the anterior tongue18 confirms the small receptive fields and high sensitivity to low-threshold forces observed psychophysically (Fig. 89-2). On the basis of their small receptive fields and low thresholds, lingual fibers could be divided into those innervating the superficial (mucosal) surface of the tongue and those innervating deeper muscle tissue. The majority of the superficial fibers were rapidly adapting—a characteristic in common with other highly sensitive structures used in exploratory activity (e.g., the hand). In contrast, the deeper receptors were all slowly adapting and may provide information about the position of the tongue.
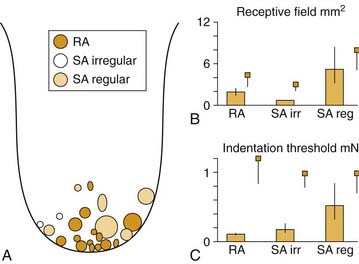
Figure 89-2. Receptive field properties of superficial mechanoreceptive afferents recorded from the human lingual nerve. A, Size and location of receptive fields of three types of mechanoreceptors: rapidly adapting (RA), slowly adapting (SA) regular, and slowly adapting irregular. B, Receptive field area. C, Receptive field threshold. Small squares indicate corresponding data from human median nerve.70 Vertical bars indicate standard error.
(From Trulsson M, Essick GK. Low-threshold mechanoreceptive afferents in the human lingual nerve. J Neurophysiol. 1997;77:737-748.)
Trigeminal endings mediating somesthetic and thermal sensitivity of the tongue and palate range from free nerve endings to an intermediate group of “semi-organized” endings19 to more highly organized endings variously referred to as Krause end bulbs,20 mucocutaneous end organs,19 or coiled terminations.21 All investigators agree that there are no Pacinian corpuscles in the oral mucosa. Based on ultrastructural criteria, Munger22 refers to many highly organized oral mucosa endings as Meissner corpuscles, similar to those found in glabrous skin of the hand. However, despite all this variation in nomenclature, many of the illustrations of the specialized endings are quite similar and show “finely wound nonmyelinated fibers” without a clearly defined capsule.19 Ultrastructural studies further reveal that some of these organized endings in the palate (but not the lingual epithelium) send axonal processes into the overlying epithelial pegs and are associated with Merkel cells.22,23 In the hand, Merkel cells are correlated physiologically with slowly adapting mechanoreceptors; however, a similar correlation has not been made in the palate, and their apparent absence in the lingual epithelium does not preclude slowly adapting mechanoreceptors in this structure (see Fig. 89-2). Thus unlike the hand, a correlation between the morphology of oral receptor endings and their response properties as rapidly or slowly adapting has not been demonstrated.
Mechanoreceptors in the PDL have been studied in some detail (see reviews by Jacobs and Steenberghe24 and Maeda and colleagues25). In addition to detecting forces directed against the teeth, PDL receptors initiate oral reflexes of jaw opening and salivation and, together with receptors in the temporomandibular joint, contribute to interdental discrimination and oral stereognosis.2,24 As many as six varieties of receptor morphology are found in the PDL, ranging from complex Ruffini-like branched endings to free nerve endings.26,27 The cell bodies for PDL receptors are located peripherally in the trigeminal ganglion and centrally in the mesencephalic trigeminal nucleus.28 Mesencephalic trigeminal innervation of the PDL is primarily in the apical region near the root and consists mostly of small, myelinated, Ruffini-like endings.29 Trigeminal ganglion innervation extends from the apical region to the more superficial region and includes small unmyelinated nerve endings.
Both rapidly and slowly adapting mechanoreceptors are found in the PDL, and it is likely that it is the location of the receptor in the ligament that determines its response characteristic. Because the tooth rotates about its fulcrum, forces directed laterally to the crown will translate to greater stretch at the root of the tooth compared with the fulcrum. Thus it is perhaps not surprising that lower-threshold fibers are found near the root and that they tend to be slowly adapting compared with receptors located near the fulcrum.30 In addition, individual Ruffini endings are not uniformly distributed around the tooth and thus display directional sensitivity to the force required to activate them. Recordings from human nerves (microneurography) demonstrate the directional sensitivity of PDL receptors31 (Fig. 89-3) and further indicate mechanical coupling between the teeth. Single fibers respond to stimulation of multiple (adjacent) teeth; however, there is no anatomic evidence that individual fibers innervate multiple teeth.32
The differential innervation of the PDL by the trigeminal ganglion and mesencephalic trigeminal nucleus has functional significance. Mesencephalic receptors are primarily medium and rapidly adapting receptor types, many with directional sensitivity. The central termination of these mesencephalic force detectors includes inhibitory connections to trigeminal jaw closer motoneurons via the supratrigeminal area.33 Thus these receptors serve a protective role in preventing potentially damaging tooth contact during mastication. In contrast, trigeminal ganglion receptors include slowly adapting mechanoreceptors (position detectors) and high-threshold C fibers (nociceptors) in addition to rapidly adapting mechanoreceptors. Because these periodontal receptors from the trigeminal ganglion terminate centrally in the sensory trigeminal complex, the source for the ascending sensory pathway to the thalamus and cortex, they provide information about tooth displacement and dental pain to the forebrain.
Although mechanoreceptors in the PDL are not encapsulated, their response characteristics are influenced by the elastic properties of the ligament. When the attachment of the ligament is compromised (e.g., during periodontitis that loosens the connective attachments of the ligament), a corresponding loss in interdental force discrimination is observed.34 Periodontal receptors also contribute to the regulation of bite force. Individuals with dentures could not bite as hard as normal dentulous subjects and could not perceive variations in their own bite force.2,35 Similar results were obtained by anesthetizing the inferior alveolar nerve.36 In contrast, anesthetizing the temporomandibular joint does not affect bite force discrimination but does impair jaw-positioning performance. Thus different populations of oral receptors may regulate sensing jaw position and controlling bite force during mastication.
Common Chemical Sense
Stimulation of the oral cavity with high concentrations of salts, acids, alkaloids, and other compounds elicits intense taste sensations but also evokes nontaste sensations ranging from stinging and burning to warm, cool, and painful. This sensitivity of the oral cavity, mediated by nonspecialized free nerve endings and shared by all mucosal membranes, is referred to as the common chemical sense or chemesthesis and should not be confused with taste. Although free nerve endings respond to many traditional gustatory stimuli, they typically display a much lower sensitivity. Electrophysiologic recordings from the lingual nerve, for example, indicate that single fibers require concentrations of sodium chloride (NaCl) 1000 times higher than those necessary to elicit a response from a gustatory fiber in the chorda tympani nerve (reviewed by Bryant and Silver37). Much lower concentrations of other types of chemical stimuli (e.g., menthol [10−4]), however, are adequate to elicit a response in trigeminal nerve fibers. The types of chemical stimuli that elicit low-threshold responses in trigeminal fibers suggest that one function of the common chemical sense is to protect the oral cavity. Responses to common chemical stimuli include reflex salivation and coughing that function to diffuse and remove offending stimuli from the mouth. The common chemical sense is not purely protective, however. Spices such as horseradish, ginger, and red pepper are effective stimuli for trigeminal afferent fibers and contribute to the flavor of food. One of the receptors for chemesthetic stimulation was recently cloned.38 A member of the TRP family of G-protein-coupled receptors, the vanilloid receptor termed “VR1” responds to both noxious heat and low concentrations of protons in addition to vanilloid compounds such as capsaicin, found in chili pepper. Stimulation of this receptor results in the opening of a cation channel, thus depolarizing the afferent fiber.
Dental Pain
Persons usually describe dental pain as either dull/burning or sharp.39 Sensations of dull burning pain are associated with stimulation of C fibers terminating in the pulp chamber, whereas sharp “bright” dental pain is associated with A-delta fiber innervation that extends a short distance into the dentinal tubules matrix interposed between the pulp chamber and the enamel covering of the tooth (reviewed in Burgess and colleagues40). Unmyelinated C fibers constitute the majority of pulpal innervation (50% to 75%); however, endings within the pulp chamber may be unmyelinated terminals of A-delta (myelinated) afferent fibers. Polymodal C fibers innervating the pulp chamber respond to thermal stimuli and, in particular, to inflammatory mediators including histamine and bradykinin, endogenous factors associated with pulp pathology. C fibers innervating the pulp chamber contain neuropeptides such as substance P and calcitonin gene-related peptide.41 The peripheral release of these neuropeptides on C fiber activation produces local vasodilation, thus increasing the pressure within the rigid pulp chamber and further augmenting C fiber activation (i.e., peripheral sensitization). The release of substance P in infected teeth has been directly measured in human patients using microdialysis, and patients with irreversible pulpitis had significantly higher levels of substance P in the pulp chamber compared with noninfected teeth.42 Although the release of neuropeptides augments pain, there is evidence that it may also reduce inflammation and promote recovery. In experiments with animals, eliminating the afferent terminal release of neuropeptides by denervating the teeth reduced wound healing after lesions were experimentally induced.43
Sharp pain is mediated by A-delta fibers extending 0.2 to 0.3 mm into the dentinal tubules encasing the pulp chamber.44 These nerve fibers respond to heat, mechanical, and osmotic stimuli applied to the distal end of the dentinal tubules that become exposed to environmental stimuli when the enamel layer is breached.45 Because the dentinal tubules are filled with a fluid, the fluid transmits mechanical, thermal, and osmotic stimuli to the proximal end of the dentinal tubules where the nerve endings are located. This “hydrodynamic” theory of dental pain has gained anatomic, physiologic, and psychophysical support and further offers an explanation of dentinal hypersensitivity. When the dentinal tubules are exposed by a cavity or other lesion, patients report sharp pain in response to innocuous stimuli such as mild temperature or osmotic stimuli (e.g., sweet compounds). However, the theory predicts that if the tubules are covered, thus limiting exposure to environmental stimuli, stimulated pain should be reduced. This had been experimentally assessed in human volunteers in whom a small cavity in a tooth was prepared (in a tooth scheduled for removal) and a conical chamber positioned over the cavity through which regulated air pressure could be delivered.46 Creating a smear layer of amorphous tooth particles in the cavity, or dissolving it away with solvents, controlled the interface between the exposed dentinal tubules and the air pressure stimulus. When the smear layer was intact, covering the dentinal tubules, it took more air pressure to induce the perception of sharp pain than when the smear layer was dissolved.
Central Projections of Trigeminal System
Afferent fibers of the trigeminal nerve enter the brainstem in the pons, bifurcate, and terminate in either the principal sensory nucleus or descend to terminate in the spinal trigeminal complex in the medulla. The bifurcation of the trigeminal nerve at the level of the pons reflects a tendency toward a segregation of function.47 In general, low-threshold mechanoreceptors predominate in the principal trigeminal sensory nucleus, indicative of a tactile discriminative function. In contrast, considerable evidence implicates the subnucleus caudalis in orofacial pain mechanisms, and many neurons in the subnucleus caudalis respond to noxious stimuli applied to the head and neck.11 These neurons include those specifically activated by noxious stimuli (nociceptive-specific neurons) and wide-dynamic-range neurons, responsive to both low- and high-intensity stimulation.
Because the receptive fields for many nociceptive neurons in the subnucleus caudalis are large and include responses to nociceptive stimuli applied to the masticatory muscles, tooth pulp, and temporomandibular joint, a role for these neurons in referred pain has been suggested.48 Anatomic studies confirm that afferent fibers innervating the oral cavity, tooth pulp, oropharynx, temporomandibular joint, masticatory muscles, and superficial skin all converge in the subnucleus caudalis.49,50 In many patients, lesions in mandibular teeth have a high likelihood of producing referred pain to the maxillary region, the cheek, and the ear, in addition to the mandible itself.51 Likewise, lesions in the maxillary teeth are often referred to the mandible, as well as the maxilla, temple, and orbital region.
In addition to subnucleus caudalis, other parts of the sensory trigeminal complex are also involved in trigeminal pain. Nociceptive responses have been obtained from extensive areas of the sensory trigeminal complex, and destruction of the subnucleus caudalis does not prevent all trigeminal pain function (reviewed in Sessle52). Case studies of patients who have undergone trigeminal tractotomy for intractable pain associated with cancer are completely analgesic on the face, but pulpal pain is intact.52 Likewise, when the principal trigeminal nucleus and subnucleus oralis were damaged after a stroke, oral and perioral pain sensitivity was diminished, as well as normal tactile sensitivity from these structures.53
Neurons in both the rostral sensory trigeminal complex (subnucleus oralis) and the subnucleus caudalis may also form a substrate for “central sensitization” in which central neurons in the pain pathway have their response characteristics magnified as a result of peripheral stimulation.54 These changes can last a variable amount of time and potentially contribute to both short-term hyperalgesia and long-term chronic pain. Fundamental to the concept of central sensitization is that neurons initially only responsive to high-threshold (nociceptor) input become responsive to low-threshold, non-nociceptive input. The increased responsiveness is thought to be mediated by A-β (non-nociceptive) input that only becomes functionally active after intense peripheral nociceptor input. One neural mechanism for the nascent response to non-nociceptive input has been studied in great detail. An intense afferent barrage of nociceptor input, following peripheral tissue damage or inflammation, “sensitizes” a central neuron via structural modification of an N-methyl-D-aspartate (NMDA) glutamate receptor. NMDA receptors are voltage sensitive and will not pass current, even in the presence of a ligand, unless the cell is sufficiently depolarized. The central release of a neuropeptide (e.g., substance P) by nociceptor afferents, however, may provide sufficient depolarization to modify NMDA glutamate receptors via intracellular signaling pathways, thereby allowing glutamate released by non-nociceptive (A-β) input to activate central neurons. A-β activation of a central nociceptor thus provides a neural mechanism for allodynia. Similar mechanisms have been demonstrated in the brainstem sensory trigeminal complex and may provide a substrate for chronic oral and facial pain.11 Experimental studies demonstrate that neuropharmacologically blocking NMDA receptors prevents temporomandibular joint or tooth pulp afferents from inducing hyperactivity in central trigeminal neurons (i.e., central sensitization).55,56
Somatosensory information reaches the ventrobasal complex of the thalamus from all major subdivisions of the trigeminal sensory complex.11 Many cells in the ventrobasal complex respond to low-intensity stimulation, indicative of a tactile discriminatory function; however, other neurons require high-intensity stimulation. The small receptive fields of both types of neurons suggest a role in localization. Other nuclei, including the posterior thalamic nuclei and the nucleus submedius, respond preferentially to high-intensity stimulation and may be involved in affective components of pain.57 Both nociceptive and non-nociceptive trigeminally activated neurons from the thalamus project to the somatosensory cortex. Electrophysiologic mapping studies in primates indicate a complex, sometimes discontinuous somatotopic map of the facial and oral region.58 In general, the face is represented medially on the cortical surface adjacent to the representation of the hand, with successively lateral representations of the teeth and tongue. Magnetic resonance imaging (MRI) in humans confirms this somatotopic representation.59
Motor Function
Oral motor functions include mastication, swallowing, respiration, and vocalization. This review will focus on mastication and the oral components of swallowing and respiration. One of the dominant concepts in oral motor physiology is central pattern generation. Chewing, swallowing, and breathing are each produced by brainstem central pattern generators that control the fundamental rate and pattern of muscle contractions that define each function. Although sensory pathways from the mouth play an intimate role in oral motor function, fundamental to the concept of central pattern generation is that afferent activity is not necessary to evoke rhythmic activity and does not provide the critical timing information for coordinated motor output.60 Although organized in the brainstem, central pattern generators for chewing, swallowing, and respiration are influenced by descending inputs from virtually all major regions of the neuraxis. Detailed reviews of oromotor central pattern generation can be found in works by Nakamura and Katakura,61 Rekling and Feldman,62 and Jean.3
Fundamental to oral motor function is the complex interplay between behaviors competing for the same muscles. Chewing, swallowing, and respiration all require the coordinated activity of masticatory, lingual, facial, and infrahyoid muscles. Swallowing and respiration further depend on pharyngeal and abdominal muscles. Motor coordination takes place on multiple levels. At a behavioral or molar level, swallowing and respiration must be coordinated to prevent aspiration of food into the airway. How this coordination is achieved is only beginning to be understood but likely involves both interactions between central pattern generators and peripheral feedback. However, individual oral motor functions also require a high level of coordination. Bolus formation during mastication requires the coordinated activity of masticatory, lingual, and facial muscles, muscles innervated by motoneuron groups highly segregated in the brainstem. Although the jaws and tongue can function independently,63 oftentimes the tongue and jaw appear inextricably “linked.”64,65 The nature of this linkage, and whether it relies on interactions between central pattern generators, reflex control, or peripheral mechanical linkage, represents a significant problem in oral motor control.
In addition to the complexity of coordination between functions and coordination between motor groups, another level of complexity in oral motor control can be found within the muscles themselves. Individual masticatory and lingual muscles are not homogeneously functioning units; muscles are oftentimes composed of multiple compartments, with muscle fibers oriented in multiple directions. Thus different parts of a muscle can be more or less active during a given behavior.66 Further adding to the complexity of oral musculature are the multiple isoforms of myosin heavy chain (MHC) proteins that form the contractile elements of the muscle fibers. The differential distribution of MHCs within different muscles and muscle compartments imparts additional degrees of freedom to motor output.
A myriad of “simpler” oral reflexes serve protective functions and contribute to complex rhythmic output. Muscle spindles in jaw-closer muscles, for example, may contribute to load regulation during chewing, and oral reflexes may assist coordination between the jaw and tongue. Autonomic oral reflexes modulate salivation and initiate digestive processes. Several recent reviews of oral reflex function are available.63,67,68
Muscles of Mastication and Reflex Control
The muscles of mastication can be divided into jaw openers and jaw closers. However, human jaw movement is more complex, even during stereotyped rhythmic mastication. During opening, the jaw translates forward; during closing it translates backward.69,70 A given muscle is not isomorphic with a single movement. The masseter, temporalis, medial pterygoid, and superior head of the lateral pterygoid muscle have major jaw-closing (mandible elevation) functions, but contraction of the masseter and lateral pterygoid protrude the mandible, whereas contraction of the temporalis muscle retracts the mandible. Contraction of the anterior belly of the digastric opens retrudes the jaw; contraction of the inferior head of the lateral pterygoid lowers and laterally directs the mandible. Contraction of the mylohyoid muscle also depresses the mandible, as does contraction of the geniohyoid muscle, a muscle innervated by the hypoglossal nucleus.
Individual muscle fibers are physiologically classified as slow (S), fast fatigue resistant (FR), or fast fatigable (FF) and correlate to a high degree with specific isoforms of MHC contractile proteins.71 Thus S fibers express the MHC-I isoform, FR units express the MHC-IIA isoform, and FF units express the MHC-IIB isoform. Individual fibers frequently contain more than one MHC isoform (i.e., hybrid isoforms),72 and overall, masticatory muscles contain a larger proportion of hybrid isoforms compared with limb and trunk muscles.73 Moreover, masticatory muscles express MHC isoforms not found in the limb and trunk, specifically MHC-fetal and MHC-cardiac-α. Thus muscle fibers in jaw-closing muscles (masseter, temporalis, and pterygoid) include numerous hybrids that expressed MHC-I combined with MHC-fetal and MHC-cardiac-α. Muscle fibers in jaw-opening muscles differ from their jaw-closing counterparts.73 Overall, jaw-opening muscles have fewer hybrid fibers and a different distribution and relative weighting of the constitutive isoforms. In the anterior (and posterior) belly of the digastric, mylohyoid, and geniohyoid muscles, there were less MHC-I, MHC-fetal, and MHC-cardiac-α compared with jaw-closing muscles, but more MHC-IIA. The presence of both MHC-fetal and MHC-cardiac-α isoforms may reflect developmental factors, but functionally, hybrid isoforms confer intermediate contraction speeds and thus greater flexibility in motor output.71
Differences in MHC isoform reflect different motor demands on jaw-opening and jaw-closing muscles. The preponderance of slow and hybrid fiber isoforms in jaw-closing muscles reflects a muscle that contracts slowly and requires flexibility against a load during mastication. In contrast, jaw opening is more ballistic and does not normally work against a load. Even the relative distribution of MHC isoforms in different muscle compartments reflects functional specialization. Jaw-closing muscles that are particularly active during mastication (e.g., the anterior temporalis and the deep masseter muscles) have more MHC-I fibers than the posterior temporalis and the superficial masseter, which are less active. The cross-sectional size of individual masticatory motor units also imparts additional degrees of freedom in muscle control. Individual efferent axons from the motor trigeminal nucleus innervate a relatively small area of the target muscle, on the order of 5%, compared with innervation patterns of the limb and trunk, which are much greater.71 Small cross-sectional innervation patterns allow specific areas of a muscle to be differentially controlled.
Jaw-opener and jaw-closer muscles differ in their investment with muscle spindles, and hence reflex function. Muscle spindles, found only in jaw-closer muscles, are involved in multiple reflexes.68,74,75 A “jaw-jerk” reflex that elevates the mandible (jaw-closing) can be elicited by a rapid depression of the mandible (i.e., tapping on the chin). This reflex, analogous to the patellar knee reflex, is mediated by muscle spindle afferents that respond to the rapid stretching of jaw-closing muscles and monosynaptically excite jaw-closing motoneurons. During mastication, these muscle spindle afferents potentially play an important role. During jaw closing against a food bolus, resistance to the load results in intrafusal fibers in the spindle that are (momentarily) shorter than the extrafusal motor fibers in which they are embedded. The consequent stretch of the spindle afferent adds excitatory drive to closer motoneurons, thus compensating for the load. This reflex action is termed the jaw-closing reflex.75
Muscle spindle afferents can also mediate a protective unloading reflex. When the jaws unexpectedly break through hard or brittle food, the rapid downward movement differentially shortens the extrafusal fibers compared with the intrafusal fibers, and muscle spindle afferent activity is decreased, thus producing a “silent period” in the jaw-closer muscle that limits excessive, potentially damaging forces directed against the teeth. These muscle spindle afferents can also indirectly potentiate jaw-opening during the unloading reflex through indirect, polysynaptic pathways. In addition to a monosynaptic excitatory synapse on jaw-closing motoneurons, these afferents can inhibit jaw-opening motoneurons through an inhibitory interneuron (Fig. 89-4). Thus during the jaw-closing phase of mastication, if there is concurrent excitation to jaw-opener motoneurons from a central pattern generator, jaw opening could be disinhibited by a lack of muscle spindle afferent input during unloading, thereby allowing the background excitation to dominate jaw-opener motoneuron activity. Damaging occlusal forces would thus be mitigated by a simultaneous lack of excitation to jaw closers and released excitation to jaw openers (see Fig. 89-4). The cell bodies for muscle spindle afferent fibers are located centrally in the mesencephalic trigeminal nucleus. Monosynaptic projections to jaw-closer motoneurons are well characterized; however, the location of inhibitory interneurons to jaw-opener motoneurons is more speculative.76
Jaw-opening muscles do not have muscle spindles; thus during the jaw-closing phase of mastication, the corresponding lengthening of the jaw-opener muscles does not itself provide an afferent signal for a reciprocal reflex. However, stimulation of mechanoreceptors located in the PDL, tongue, and other soft tissues of the mouth can initiate reflexive jaw opening, at least in many nonprimate mammals.68,74,75 The jaw-opening reflex is, at a minimum, disynaptic through neurons in the trigeminal sensory complex and may well involve additional interneurons (Fig. 89-5). Although the reflex can be elicited by non-noxious stimulation, it is generally thought to serve a protective function by protecting soft tissues (e.g., the tongue) against potentially damaging occlusal forces. The existence of a jaw-opening reflex in humans is still in doubt. Although it cannot be as readily demonstrated with sensory stimuli sufficient to produce it in experimental animals, robust electrical stimulation delivered to the upper lip produces electromyographic (EMG) activity in the anterior digastric muscle of humans.76 It is of long latency, consistent with a polysynaptic substrate as suggested by animal studies.
Lingual Muscles and Reflexes
The tongue is composed of both intrinsic and extrinsic muscles innervated by the hypoglossal nerve (reviewed in Travers77). Extrinsic lingual muscles include the major tongue protruder muscle, genioglossus, major tongue retractor muscles, styloglossus and hyoglossus, as well as palatoglossus muscle. Intrinsic muscles of the tongue consist of the vertical, transverse, superior, and inferior longitudinal muscles. The geniohyoid often functions with the lingual muscles during tongue protrusion.78,79 Most lingual movements involve both extrinsic and intrinsic muscles. The hydrostatic model of lingual function, in which the tongue is modeled as a closed bag, postulates that during tongue protrusion by contraction of the extrinsic genioglossus and geniohyoid muscles, the tongue is further lengthened by the simultaneous contraction of the intrinsic vertical and horizontal intrinsic muscles.80 Likewise, shortening of the tongue during retraction is augmented by contraction of the longitudinal muscles together with the extrinsic hyoglossus and styloglossus muscles. Coactivation of different combinations of intrinsic muscles can curl or deviate the tongue.
Expression of different MHC isoforms varies across different human lingual muscles.73,81,82 Intrinsic muscles of the anterior tongue have a large proportion of type MHC-IIA fast fibers in contrast to the posterior tongue, in which MHC-I (slow) and hybrid MHCs predominate. The geniohyoid, as with other suprahyoid muscles, has a large proportion of MHC-I fibers. The distribution of type II (fast) fibers in the anterior tongue is consistent with a role in fast, flexible movements compared with posterior tongue activity.
Although lingual muscles contain muscle spindles,63,83,84 it is unclear whether there are any monosynaptic inputs from sensory afferents onto hypoglossal motoneurons (reviewed in Travers77). Rather, muscle spindle afferents travel in the ansa cervicalis and hypoglossal nerve and terminate in either the sensory trigeminal complex or the nucleus of the solitary tract. Electrical stimulation of the hypoglossal nerve elicits synaptic responses in hypoglossal motoneurons, as well as facial85 and trigeminal motoneurons.86 Lingual reflexes can also be elicited by stimulation of virtually any of the afferent nerves innervating the oral cavity. Depending on the site of stimulation, either a protrusive or retractive movement of the tongue is produced. An overview by Lowe87 on the clinical significance of lingual reflexes emphasizes a protective role, either for the tongue itself during mastication or for the airway during swallowing.
Compounding the complexity of interpreting lingual reflexes are observations that reflex excitation of the tongue rarely influences a single lingual muscle, and contraction of a single lingual muscle can move the tongue in more than one plane.88 For example, although a primarily retrusive movement of the tongue is produced by electrical stimulation of the lingual nerve, both protruder and retractor hypoglossal motoneurons are excited. Electrical stimulation of the glossopharyngeal nerve that innervates mechanoreceptors on the posterior aspect of the tongue and oropharynx also elicits tongue movement. Similar to the lingual nerve, stimulation of the glossopharyngeal nerve excites both protruder and retractor motoneurons, and the movement of the tongue is primarily retrusive. The simultaneous activation of the glossopharyngeal nerve afferent fibers by electrical stimulation, however, may mask a more complex reflex organization. Lowe has suggested that stimulation of lingual receptors innervated by the glossopharyngeal nerve elicits a primarily retrusive movement of the tongue, in contrast to the lingual protrusion produced by stimulating pharyngeal regions innervated by the glossopharyngeal nerve.63 Thus both lingual and glossopharyngeal reflexes may protect the tongue during the occlusal phase of mastication with a retrusive movement.
In contrast, electrical stimulation of the superior laryngeal nerve that innervates laryngeal mechanoreceptors depolarizes protruder motoneurons and produces a protrusive action of the tongue. Thus mechanoreceptors in the oropharynx and larynx innervated by the superior laryngeal and the glossopharyngeal nerve may preserve airway patency during a swallow with a protrusive tongue movement. Lingual reflexes also play a protective role in respiration. When normal respiration is impeded by hypoxia, a normal breathing pattern, eupnoea, is replaced by gasps.88,89 Gasps are associated with the coactivation of lingual protruder and retractor muscles that enlarge the upper airway.90–92
Jaw-Tongue Reflexes
Oromotor reflexes can involve multiple motor systems. Electrical stimulation of either the masseteric or anterior digastric nerves, for example, suppresses genioglossus activity, suggesting that proprioceptive or nociceptive signals from the trigeminal musculature inhibit lingual protrusion.93 In contrast, passively depressing the mandible (in cats) excites the genioglossus muscle, suggesting that lingual protrusion may be reflexively assisted during jaw opening when the tongue is not subject to occlusal force.94 Further evidence that masticatory muscle proprioceptive afferents influence hypoglossal motoneuron activity comes from experimental lesions of Probst’s tract. Ishiwata and colleagues95 showed that such lesions, which destroy descending mesencephalic projections, suppressed hypoglossal activity induced by passive jaw opening but left intact hypoglossal activity induced by stimulating a cutaneous oral sensory nerve. A jaw-tongue reflex in humans may also be mediated by masticatory muscle proprioceptive afferents.96 Stimulation of the hypoglossal nerve, which contains some afferent fibers, inhibited the masseteric (jaw-closing) reflex.86
Autonomic Reflexes
In addition to somatomotor reflexes, stimulation of the oral cavity elicits numerous autonomic responses. Gustatory and mechanical stimuli are highly effective in eliciting the flow of saliva during mastication.97 The stimulation of receptors in the PDL may be one source for reflex salivation. In both rabbits and humans, there is a high correlation between parotid flow and mandibular movement, especially on the working, ipsilateral side. In humans, selective anesthetization of the nerves innervating the PDL significantly reduced the amount of saliva elicited from crushing a food stimulus held between the teeth.98
Both location and stimulus modality influence the release of saliva.97,99 Stimulating the anterior part of the tongue is most effective for evoking salivation from the sublingual and submandibular glands, but posterior tongue stimulation is more effective for producing parotid gland flow. Aversive gustatory stimuli such as (sour) acids or (bitter) quinine hydrochloride are more effective for eliciting saliva than stimulation with weak salt or sucrose solutions. In animal experiments, sweet stimuli were the most effective stimuli for the release of the enzyme amylase from the parotid gland.100
Mechanical and chemical stimulation of the oral cavity also initiate the release of digestive enzymes. These cephalic phase responses include the release of gastric acid, insulin, glucagon, and pancreatic polypeptide.101,102 An increase in gastric motility and emptying of the gallbladder also occurs. Although cephalic phase insulin release (CPIR) is a highly variable response not occurring in all individuals, insulin levels rise, on average, 25% above baseline within 2 minutes of oral stimulation. This release is neurally mediated because it does not occur in the absence of an intact vagus nerve.103 In animal studies, sweet stimuli—particularly glucose—are most effective in triggering CPIR,104,105 but are somewhat less effective in humans (discussed by Teff102). Rather, “palatable” stimuli appear more effective in general. Although cephalic phase insulin release accounts for perhaps only 1% of the total insulin release associated with a meal, this amount underestimates its potential importance in glucose metabolism. Experimental studies in which CPIR is bypassed by intragastric infusions can result in both hyperinsulinemia and hyperglycemia.106,107 One possible mechanism for this is that vagally mediated insulin release acts as a signal on hepatic receptors to further regulate glucose metabolism; that is, it is acting as a signal to initiate metabolic events rather than simply to convert glucose.102 Other cephalic phase responses represent larger fractions of total meal responses. In humans, cephalic phase gastric acid secretion can reach 50% of total meal release, antral motor activity can reach 70% of that achieved during a meal, and gallbladder emptying can reach 50%.101
Although cognitive factors and other sensory stimuli such as sight and sound can elicit cephalic phase responses, oral stimuli are usually the most effective. Oral stimuli signals carried by the gustatory and trigeminal nerves influence preganglionic parasympathetic vagal neurons located in the dorsal motor nucleus of the vagus.108 Oropharyngeal receptors innervated by the superior laryngeal nerve may also influence digestive functions.108 There is increased diuresis in response to drinking a saline solution as compared with the intragastric infusion of the same volume of fluid.109
Mastication
Food consumption through the oral cavity can be characterized as a series of stages or phases (Fig. 89-6). Different stages of ingestion have been defined by placing small metal markers in the jaws, hyoid, and tongue. These markers can be detected with high-speed cine-fluorographic techniques, allowing the movements of the internal oral apparatus to be monitored during the entire ingestive sequence of the awake preparation. The division of feeding into five dynamic stages by Hiiemae and Crompton64 is indicated on the second tier of Figure 89-6. The first stage of putting food into the mouth (ingestion) is followed by intraoral transport and the positioning of food between the molars (second stage) for mastication (third stage). Intraoral transport to the back of the tongue (fourth stage) initiates deglutition (fifth stage). The duration of each stage of feeding is highly species specific and variable, depending on what is being ingested. Fluid consumption does not require mechanical breakdown by mastication and thus has only three stages. In humans, drinking uses the same muscles as mastication, but the coupling among the facial, trigeminal, and lingual muscles is different. The orbicularis oris muscle contracts to form a tight seal during human drinking (sucking) but relaxes during mastication.
The movements of mastication can be further subdivided. Kinematic measurements during mastication indicate that rhythmic masticatory movements of solid food typically involve several distinct components.110,111 Beginning the masticatory cycle with an open mandible, the jaw closes rapidly and then more slowly. The transition from fast closure to slow closure occurs when the teeth make contact with solid food and is thought to involve sensory feedback from the PDL. More detailed analysis of the opening phase of mastication indicates additional complexity. After the slow-closure phase, when the teeth make maximal intercuspation, the masticatory cycle continues with a slow-opening phase followed by a fast-opening phase.
Feeding sequences analyzed using combined sirognathography and electromyography or video-fluorography present a modified picture of the human ingestive sequence.112,113 Human jaw movements associated with eating natural foods do not show obvious changes in the rate of opening and closing during rhythmic mastication. Thus the stages of fast closing, slow closing, and slow opening were not evident as in animal studies. Nevertheless, the ingestive sequence could be divided into three stages, beginning with biting and transport of the bolus to the molars, chewing, and “clearance” (swallowing). A distinct stage from chewing to bolus formation for swallowing was not obvious, and swallowing occurred during mastication and as a terminal event.
Although mastication involves coordinated activity of the jaws, hyoid apparatus, and tongue,64 the majority of electromyographic studies of mastication have focused on the jaw musculature. Jaw opening during mastication is associated with activity in the anterior digastric muscles and the inferior head of the lateral pterygoid muscle.111,114 The closing phase of mastication begins with contraction of the masseter muscle, followed by the temporalis, medial pterygoid, and superior head of the pterygoid, which are recruited during the power stroke (slow closure). Food is typically chewed unilaterally. Although the trigeminal musculature is bilaterally activated during mastication, the ipsilateral (working) side is active earlier.
Food consistency is one factor affecting the masticatory rhythm. In a study of the effects of hardness on chewing, Plesh and colleagues115 observed that most subjects chewed hard gum at a slightly slower rate than soft gum. The decreased frequency of chewing was associated with significantly longer opening and occlusal phases of chewing rather than with the closing phase, despite the significantly greater EMG activity in the masseter muscle. Age is another factor that affects the masticatory rhythm.116 Older subjects chewed at the same frequency as younger subjects (approximately 1.4 Hz), but the structure of the rhythm was different. The older subjects opened and closed their mouths at a slower velocity but achieved the same overall chewing rate by not opening their mouths as far. Movement irregularities during chewing were also observed during the jaw-opening and jaw-closing phases of mastication in patients diagnosed with temporomandibular pain.117 Unlike the smooth, uninterrupted alteration between opening and closing seen in healthy persons, patients with temporomandibular pain frequently started reopening their mouths during the closing phase of mastication or reclosed during the opening phase.
Experimental studies indicate that the masticatory rhythm is centrally programmed; that is, a peripheral stimulus is not necessary to initiate the masticatory rhythm, nor is feedback from the active muscles necessary to sustain the response.114 Fictive mastication evoked by central stimulation in a paralyzed experimental preparation indicates that neither the afferent limb of the jaw-opening reflex nor that of the jaw-closing reflex is necessary to generate the masticatory rhythm. Thus the alternating activation of a jaw-opening reflex followed by a jaw-closing reflex does not explain the origins of the masticatory rhythm.
Nevertheless, both the jaw-opening and jaw-closing reflexes are functionally entwined in rhythmic oral behavior, and the excitability of these reflexes varies as a function of jaw position during rhythmic opening and closing.118 In general, the jaw-opening reflex is attenuated during rhythmic masticatory movements as compared with a stationary mandible. In particular, low-threshold mechanical stimuli are less effective than high-threshold stimuli in producing a jaw-opening reflex when applied during rhythmic masticatory movements. Thus during the occlusal phase of mastication, a protective jaw-opening reflex can be initiated in the presence of unexpected mechanical forces directed against the teeth or soft tissues, but innocuous mechanical stimulation associated with chewing will not interrupt the masticatory rhythm.
Transection studies relying on electrical stimulation to induce fictive jaw movements localized the central pattern generator for mastication to the medial core of the reticular formation. More recent studies using reversible pharmacologic lesion techniques in awake, freely moving (feeding) animal preparations indicate that a necessary substrate for rhythmic lingual/masticatory movements is in the lateral reticular formation in a region overlapping with substantial populations of preoromotor interneurons.119,120 This region of the brainstem reticular formation is also the target of descending projections from metabolic integrative substrates in the hypothalamus and from the motor cortex.61,121
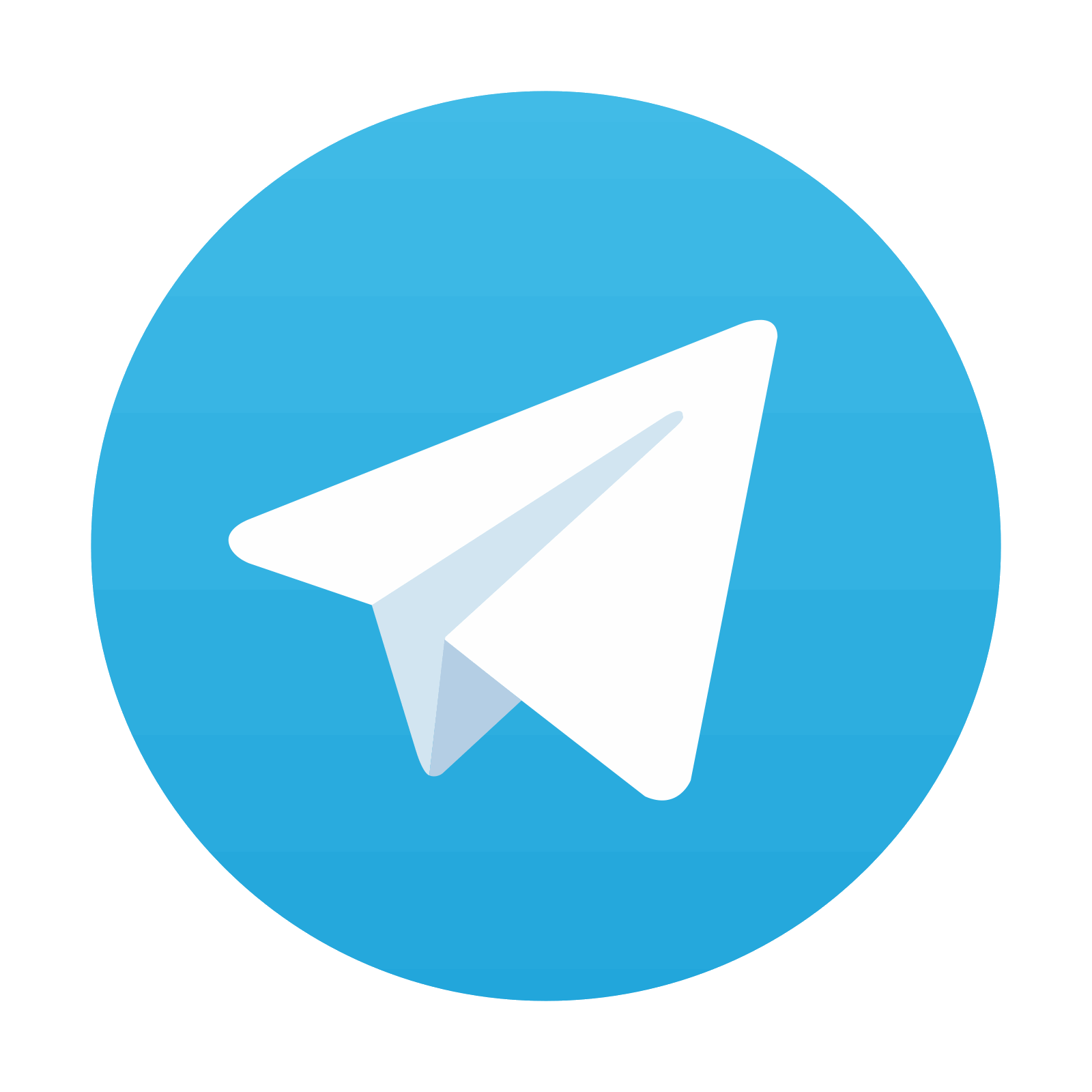
Stay updated, free articles. Join our Telegram channel
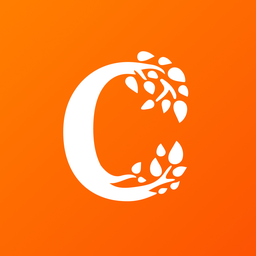
Full access? Get Clinical Tree
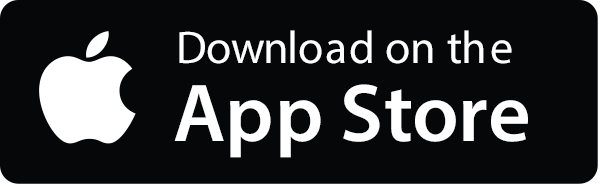
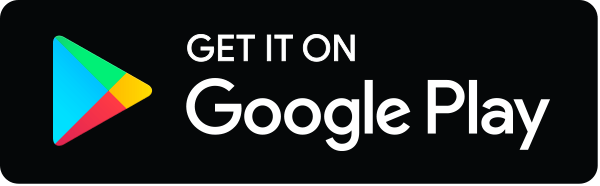