Li et al. 2009 [3]
Jiao et al. 2009 [2]
Jiao et al. 2010 [17]
Zhang et al. 2011 [8]
Yang et al. 2011 [6]
Zhang et al. 2012 [7]
Blatter et al. [18]
Lee et al. 2013 [9]
Xi et al. 2013 [10]
PA
Excitation λ
532 nm Nd:YAG (Elforlight SPOT)
532 nm Nd:YLF (Edge-Wave IS8II-E) pumped 580 nm dye (Sirah GmbH Cobra)
532 nm Nd:YAG (Elforlight SPOT-10-100-532)
355 nm Nd:YAG pumped OPO (Newport Spectra-Physics), output 410–2,100 nm (used 670 and 680 nm)
532 nm Nd:YAG pumped Ti:sapphire (Symphonics-TII, LS-2122)
532 nm Nd:YAG (Elforlight SPOT-10-100-532) pumped dye laser (λo = 580 nm, Δλ = 20 nm)
532 nm Nd:YAG (Coherent Corona)
1,064 nm Nd:YAG microchip (Teem Photonics SNP-20 F-100) coupled into PCF (output 600–1,700 nm)
532 nm Nd:YAG
Excitation pulse
1.2 ns
6 ns
2 ns
8 ns
20 ns
2 ns
250 ns
0.7 ns
Not reported
Excitation pulse repetition rate
Up to 20 kHz possible
1,024 Hz
Up to 30 kHz possible
50 Hz
15 Hz
Up to 30 kHz possible
5 kHz
21 kHz
10 Hz
Detection configuration
Forward
Backward
Backward
Backward
Backward
Forward
Backward
Forward
Backward
Transducer
75 MHz center frequency (−6 dB), focused (Olympus NDT V2022)
15 MHz, bandwidth 80 %, active element diameter 6 mm unfocused (Olympus NDT V313)
30 MHz bandwidth 50 %, active element diameter 1.0 mm unfocused needle (Custom)
39 MHz (−3 dB) FPI sensor with 1,550 nm interrogation laser (Custom)
35 MHz center frequency (−6 dB), unfocused (Olympus NDT Panametrics 5900PR)
30 MHz, bandwidth 50 %, active element diameter 0.4 mm unfocused needle (Custom)
Phase-sensitive OCT
30 MHz center frequency, acoustic bandwidth of ∼ 18 MHz
10 MHz unfocused
Axial resolution
14 μm
50 μm
23 μm
20 μm
∼55 μm
23 μm
∼375 μm
74.5 μm
Not reported
OCT
Detection
Spectrometer/CCD
Spectrometer/CCD
Spectrometer/CCD
Spectrometer/CCD
Swept-source/DBD
Spectrometer/CCD
Swept-source/DBD
Spectrometer/CCD
PD/RSOD line
Light source
λo = 829 nm, Δλ = 36.4 nm SLD (InPhenix, IPSDD0803)
λo = 840 nm, Δλ = 50 nm SLD (Superlum)
λo = 870 nm, Δλ = 100 nm SLD (InPhenix)
λo = 1,050 nm, Δλ = 70 nm ASE (NP Photonics)
λo = 1,310 nm, Δλ = 110 nm (Santec HSL-2000)
Same as PA
λo = 1,310 nm FDML laser, 120 nm full bandwidth
Same as PA
λo = 1,310 nm, Δλ = 75 nm SLD (DenseLight, DL-BX9-CS3159A)
Axial resolution
5.9 μm (tissue)
6 μm (tissue)
4 μm (tissue)
8 μm (tissue)
12 μm (air)
9.5 μm (air)
∼12 μm (air)
14.5 μm
10 μm (air)
PA/OCT
Lateral resolution
5 μm
20 μm (OCT)
20 μm
18 μm (OCT)
25 μm (OCT)
Not reported
Not reported
12 μm (OCT)
15 μm
7.8 μm (PA)
50–100 μm (PA)
∼450 μm (PA)
11.5 μm (PA)
Scanning
2D moving objective on translation stage
2D galvanometer scanning
2D galvanometer scanning
2D galvanometer scanning
Probe translated mechanically 1D
2D galvanometer scanning
Sample moving via 2D translation stage
Sample moving via 2D translation stage
Probe on 2D mechanical stage
Scan rate
∼1 kHz
1,024 Hz
24 kHz
47 kHz (OCT)
20 kHz(OCT)
5 kHz
5 kHz
Not reported
1 kHz (OCT)
50 Hz (PA)
15 Hz (PA)
10 Hz (PA)
Simultaneous imaging?
No
Yes
Yes
No
No
Yes
Yes
No
No
In vivo application
Mouse ear
Mouse ear
Rat retina
Mouse and human skin
N/A
Mouse ear
N/A
N/A
Mouse ear
52.1.3.1 PAM/OCT
Li et al. demonstrated the use of OR-PAM/OCT for evaluating microcirculation in the mouse ear in 2009 [3]. The depth range was estimated to be 1.5 mm for OR-PAM, which was comparable to the 1.8 mm depth for OCT. While this was the first effort to show the feasibility of a multimodal combination with OCT, the transmission (forward) mode configuration required the sample to be very thin. In addition, a mechanically translated objective meant very slow acquisition time (2.5 frames/s), which further limits the application of this configuration. Despite these restrictions, the system was later used to look at neovascularization of a tissue scaffold in anesthetized animals, with the relatively thin mouse ear again used as a model [1].
An effort by Jiao et al. in 2009 looked at the microvasculature of the mouse ear using backward mode detection with an unfocused 15 MHz ultrasound transducer [2]. The authors presented synchronized simultaneous acquisition, though the measurement rate was limited by the excitation pulse repetition rate of the PAM system to 1,024 Hz, whereas a 24 kHz A-scan acquisition would have been possible given their OCT CCD integration time. Liu et al. applied this system for spectroscopic measurements (at 570 nm, 578 nm, 588 nm, and 590 nm) to measure oxygen saturation (sO2) with a custom-built unfocused 35 MHz needle transducer [4]. They used these sO2 levels together with Doppler OCT blood flow velocity and total hemoglobin concentration (from laboratory-based blood tests) to measure the metabolic rate in the mouse ear.
52.1.3.2 PAM/OCT for Ophthalmic Applications
Because one of the most common clinical applications of OCT imaging is in ophthalmology, a natural extension of multimodal PAM/OCT imaging is for in vivo retinal imaging. Access to absorption-based contrast could be used to evaluate the retinal pigment epithelium in diseases like age-related macular degeneration, and sO2 measurements might be used to assess blood vessel status in diabetic retinopathy. Jiao et al. presented a combined PAM/OCT ophthalmoscope system that used an unfocused transducer placed directly on the sclera to acquire preliminary in vivo images of the rat retina at a 93 Hz frame rate (256 A-scans/frame; volumetric image in 2.7 s) [17]. The pulse energy of the excitation laser source was 10 μJ at the output of the laser and estimated to be less than 40 nJ/pulse at the sample. In a study published in 2012 by Song et al., this PAM/OCT system was further extended to include additional modalities like scanning laser ophthalmoscopy and fluorescein angiography and again demonstrated in the rat retina [5]. Despite these preliminary demonstrations in animals, it is unclear whether the pulse energy necessary to generate a PA signal is within the maximum permissible exposure limitation for the human retina.
52.1.3.3 PAM/OCT with a Single Light Source
Using a single light source for PAM excitation and OCT imaging would have several advantages including increased portability and simpler co-registration and synchronization. The first demonstration of a single pulsed light source for PAM/OCT by Zhang et al. (2012) is promising but has some important limitations [7]. Because the same light source and delivery system were used, the images were laterally co-registered. However, the source operates in the visible light range (λo = 580 nm, Δλ = 20 nm), where exposure limits are lower than at near-infrared (NIR) wavelengths and there is shallower penetration into tissue. In addition, the spectrum was very noisy and the quality of the OCT images suffered as a result. The source was also not operated at the maximum pulse repetition rate (30 kHz) but instead at 5 kHz. Considering these factors and the limited source bandwidth and axial resolution, this solution is suboptimal for most biological applications of OCT.
Lee et al. have presented PAM/OCT imaging of black and white human hair using a single nanosecond-pulsed supercontinuum NIR laser source [9]. The output range was 600–1,700 nm, though the spectrometer limited detection to between 650 and 900 nm, with the dominant wavelength band beyond 1,064 nm, making this wavelength range more appropriate for OCT imaging. However, many endogenous molecules that would provide spectroscopic contrast for PA imaging, such as hemoglobin, do not exhibit maximum absorb in this wavelength range. Furthermore, the forward mode configuration for PAM detection would limit any potential in vivo applications to thin samples, and the sample is translated mechanically, limiting the imaging speed.
52.1.3.4 Endoscopic Delivery
A step towards intraoperative PA and OCT imaging was made by Yang et al., who developed a miniaturized probe for endoscopic measurements of PAM/OCT signals along with conventional pulse-echo ultrasound [6]. The excitation pulse repetition rate was 15 Hz, which limited the speed of acquisition, but they presented sequential imaging of ex vivo of ovarian tissues at 740 nm with excitation energy of 1 mJ/pulse. The endoscope had a 5 mm diameter. Xi et al. also recently demonstrated PAM/OCT detection using a smaller endoscopic delivery probe with a diameter of 2.3 mm. Their system consisted of a low-frequency unfocused 10 Hz transducer with time-domain OCT detection at 1 kHz [10]. If scanning speed can be improved, these delivery systems may further extend the clinical utility of multimodal PA/OCT imaging.
52.1.3.5 All-Optical Detection
A major drawback to several of the multimodal approaches presented above – and the reason many of them have only been demonstrated in the mouse ear – is their forward mode detection configuration. Backward mode optical detection methods may eventually offer a more practical alternative with respect to clinical translation.
Noncontact photoacoustic sensing by optically probing tissue has recently been demonstrated [18–24]. These methods optically detect the displacement of tissue that occurs upon an acoustic wave reaching the surface. Especially promising for combination with OCT are approaches that use low-coherence interferometry (LCI) for acoustic sensing. Wang et al. presented a method for PAM using homodyne LCI detection of surface displacement [24]. This approach, however, is very sensitive to ambient vibrations, which can change the optical path length more than the path length change that is expected from a PA disturbance. To account for this, the authors synchronized acquisition to the point at which the interferometer is most sensitive. One drawback to their approach is that their configuration only allows for combination with time-domain OCT detection, limiting their acquisition speed. The approach by Blatter et al. demonstrated simultaneous PA/OCT imaging using phase-sensitive swept-source OCT, which could potentially be much faster [18]. Currently, however, their implementation is limited to mechanically scanning the sample.
The next section (Sect. 52.2) describes the transduction of PA signals using an optical detection mechanism based on an FPI sensor and its combination with OCT as presented by Zhang et al. in 2011 [25]. To date, this is the only method to have been applied for in vivo human imaging.
52.2 Multimodal All-Optical PAT and OCT Imaging with Fabry-Perot Sensor
Beard and colleagues first described the fundamental principles of backward mode ultrasonic detection using a FPI sensor with an interrogation laser in 2004 [26]. There are multiple advantages associated with this optical approach over conventional sensing techniques for multimodal imaging. The sensor:
Is interrogated using a laser in backward mode configuration, which means it is not necessary for the sample to be very thin
Can be designed to have a transmission window that allows for combination with OCT at 1,050 nm
This section describes sensor transduction of ultrasonic signals in the context of one implementation of a PAT system combined with a spectral-domain OCT system at 1,050 nm [8].
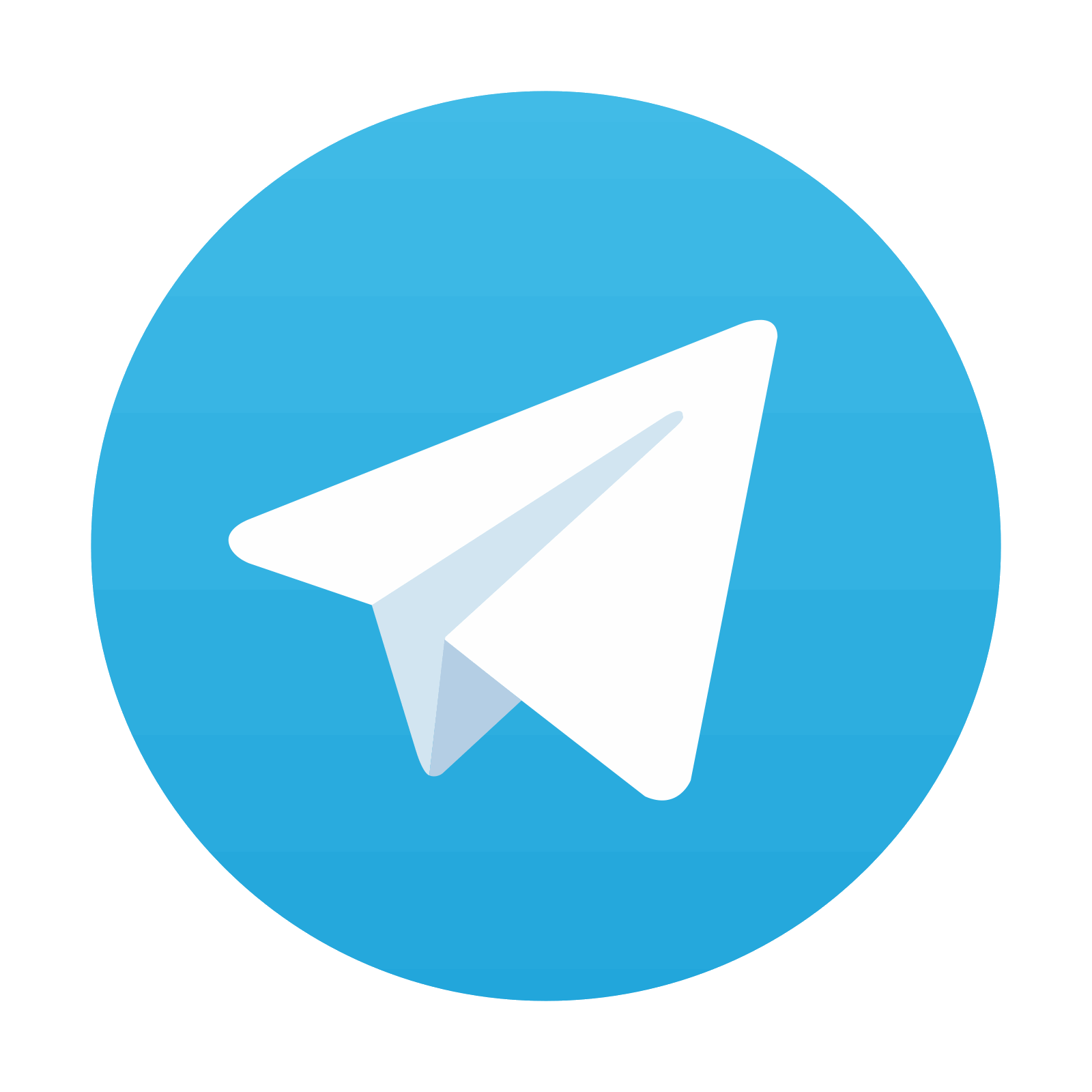
Stay updated, free articles. Join our Telegram channel
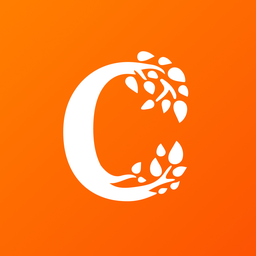
Full access? Get Clinical Tree
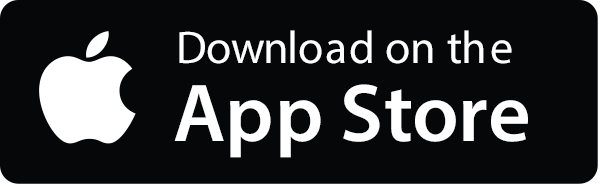
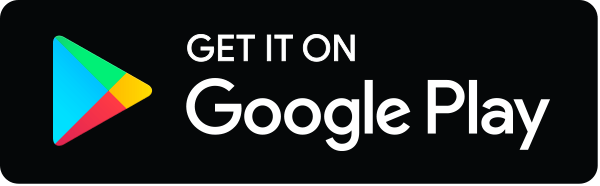