Fig. 11.1
Summary of oxidative stress defense system in eye lens. Multiple exogenous (ultraviolet light, drugs, metals, and smoke) and endogenous (peroxisomes, lipooxygenases, immune activity and altered mitochondrial respiration) sources of oxidative stress result in the formation of multiple forms of ROS such as O2·−, H2O2, and •OH. Two types of antioxidant systems (general and enzymatic detoxifiers) normally detoxify these reactive oxygen species to maintain lens homeostasis. These include GSH, MnSOD, CuZnSOD, catalase, the peroxiredoxins, and glutathioine peroxidase. Simultaneously, metallothioneins and ferritin function to limit access to free metals. Failure of these antioxidant systems leads to oxidation of proteins, loss of protein function, and protein aggregation. Specific repair systems including the Msrs and thioltransferase, thioredoxin can repair proteins restoring their normal function. Chaperone proteins such as αA-crystallin, αB-crystallin, and Hsp27 can remove toxic protein aggregates. Failure of these antioxidant defense and repair systems results in irreversible protein aggregation, loss of lens cell function, and ultimately cataract formation. ROS Reactive oxygen species, GSH glutathione, MnSOD Manganese superoxide dismutase, CuZnSOD copper zinc superoxide dismutase, Msrs methionine sulfoxide reductases, Hsp27 Heat shock protein 27
11.1.2.3 Oxidative Stress Defense and Repair Systems of the Ocular Lens
General ROS Scavengers of the Ocular Lens
General ROS scavengers can be roughly described as those molecules that nonenzymatically eliminate ROS by acting as chain breakers to directly prevent the propagation of ROS formation through direct binding and detoxification of ROS and/or ROS generators. In the lens, the primary reducing system or ROS scavenger is glutathione (GSH). Other important and well studied ROS scavengers in the lens are ascorbate (vitamin c) and vitamin E, while the cartotenoids, which are supplied solely in the diet, have been the subject of multiple supplementation studies to determine their ability to prevent or delay AMD and cataract.
Glutathione
GSH is the primary scavenger of ROS in the lens and the predominant reducing system in other ocular tissues, including the retina and cornea where it protects against chemical and oxidative stress-induced damage [33]. GSH is a dual function peptide that also participates in regulation of DNA and protein synthesis, cell-cycle control, signal transduction, and proteolysis. It is also involved in multiple metabolic pathways and the immune response [34]. GSH exists in unusually high levels in the lens. GSH functions to maintain protein thiol groups in their reduced form and therefore maintaining normal protein function [33]. GSH is maintained in its reduced form by the enzyme glutathione reductase. Under normal cellular conditions in the lens epithelium [35, 36] GSH is almost entirely found in its reduced state [37, 38] with barely detectable levels of the oxidized form of GSH (GSSG). GSH is also found at high levels in the lens fiber cells where it is likely transported from the epithelium to the fiber cells through lens connexins. GSH is completely broken down and resynthesized in the rabbit lens about every 48 h [39]. The epithelium of the lens also contains an active glutathione redox cycle. This pathway involves glutathione reductase (GSH-Rx), NADPH, and the hexose monophosphate shunt (HMPS) which all function to reduce GSSG back to active GSH [40–42]. Cultured human lens cells challenged with H2O2 show a linear increase in HMPS activity while rabbit lenses subjected to t-butyl hydroperoxide (tBHP) stress show near complete oxidation of GSH to GSSG, but this ratio is reversed after recovery in complete medium [43] possibly as a consequence of increased shunt and glutathione redox cycle activity. Treatment of cultured lens epithelial cells with 0.1 mM H2O2 for up to 180 min showed depletion of the GSH pool over the first 60 min followed by full recovery [44], illustrating that lens cells react quickly to reduced GSH levels. Levels of reduced GSH have been shown to decrease in the human lens with increased aging [35] [45, 46] and upon cataract formation [36]. GSH was also observed to decrease in normal guinea pig lenses with aging, while slight decreases in guinea pig lens GSH were found following hyperbaric oxygen treatment [47]. Decreased concentrations of GSH or a shift from GSH to GSSG in the lens is believed to increase the rate of posttranslational modifications of crystallins [48], and to perpetuate damage to key proteins containing –SH groups including Na/K-ATPase, cytoskeletal proteins, and proteins associated with membrane permeability [43].
Ascorbate
Ascorbate (vitamin C) is found at high levels in the lens and is believed to be capable of consuming oxygen in the lens [49]. In the presence of redox available metal ions, ascorbate is oxidized to dehydroascorbate and H2O2 and in the process O2 is consumed [10]. Dehydroascorbate is subsequently reduced back to ascorbate with reduced GSH and/or NADPH [50]. Ascorbate is transported from the plasma across the blood-aqueous barrier by the ciliary body into the aqueous humor where it is believed to supply the rest of the eye [51]. In addition to its oxidative role in H2O2 production, ascorbate can also act as a strong reductant and scavenger of ROS, particularly O2·−. Ascorbate decreases membrane damage in diabetic rats [52], and photoperoxidation of lens membranes [53]. It also prevents riboflavin mediated light-induced damage to cation pumps in the lens [54, 55] and it plays a role in lens development and maintenance of transparency during development [56]. Ascorbate has been shown to protect the rat lens against selenite-induced cataract [57]. Incubation of mouse lenses with high levels of ascorbate also protected against ROS-mediated decreases in membrane transport, ATP production, and decreased GSH levels [58]. It is known that measured levels of ascorbate decline with age, possibly contributing to cataract formation. In fact, the ratio of ascorbate to dehydroascorbate decreased in cataractous lenses compared to clear lenses [59, 60], suggesting a requirement for ascorbate in lens homeostasis and prevention of cataract. These data provide evidence for a protective role for ascorbate in the lens, however, oxidized ascorbate–dehydroascorbate is likely damaging to the lens, suggesting that ascorbate can also contribute to lens oxidation and cataract formation, for instance, dehydroascorbate causes precipitation and cross-linking of bovine lens crystallins in vitro [61]. The interaction of ascorbate with light in the presence of metal ions, as described above, leads to the formation of H2O2 and subsequent interaction of H2O2 with metal ions, particularly Iron (Fe) that could produce the damaging species •OH. In this way ascorbate can actually become a pro-oxidant and lead to protein damage via both H2O2 and Fenton production of •OH. The addition of both Fe and ascorbate to cultured lens epithelial cells led to a significant increase in ferritin synthesis (the intracellular iron-storage protein) [62] and increased Fe loading into ferritin [63], therefore protecting against oxidative damage. A number of studies (reviewed by Chui & Taylor [64]) indicate that consuming elevated levels of antioxidants such as ascorbate, carotenoids, and tocopherol is associated with delayed development of various forms of cataract. Indeed the Blue Mountains Eye Study found that participants with the highest quintile of total intake of vitamin C had a reduced risk of incident nuclear cataract [65]. Even more effective was an above-median intake of combined antioxidants (vitamins C and E, β-carotene, and zinc) which was also associated with a reduced risk of incident nuclear cataract [65].
Vitamin E
Vitamin E, the most important fat soluble antioxidant, is proposed to be an important chain breaking antioxidant for prevention of lipid peroxidation. Vitamin E is actually an umbrella term for a group of compounds called tocopherols and tocotrienols. α-tocopherol, the most active naturally occurring form, contains a chromane ring which reacts with organic peroxyl radicals and accounts for its antioxidant activity. It protects tissue lipids from free radical attack [66]. There is evidence to suggest that vitamin c acts to recycle the α-tocopheryl radical back to α-tocopherol, therefore implying a synergistic function between these two antioxidants [50]. Vitamin E supplementation has been shown to protect rat lenses against radiation-induced cataract [67]. The delaying or preventive effect of vitamin E on cataractogenesis has also been studied in in vivo sugar cataract models, for example galactose cataracts. Two studies showed that vitamin E supplementation showed no protective effect on galactosemic cataract in rat lenses [68, 69] but two other studies showed that direct topical instillation of vitamin E liposomes into eyes increased rat lens vitamin E and helped protect against galactosemic cataract [70, 71]. Just as the animal models for vitamin E supplementation and cataractogenesis produce mixed results so do the many human trials examining vitamin E supplementation and/or dietary/plasma levels and their association with cataract. Levels of lutein-zeaxanthin, retinoid, or α-tocopherol showed no differences between normal and cataractous human lenses [72] but in regular users of multivitamin supplements, the risk of nuclear opacification was reduced by one third. In regular users of vitamin E supplements and persons with higher plasma levels of vitamin E, the risk was reduced by approximately half [73]. A low plasma vitamin E level was associated with a 3.7-fold excess risk of the progression of early cortical lens opacities compared with the highest quartile in hypercholesterolemic eastern Finnish men [74]. In the Beaver Dam Eye Study, persons in with higher total serum tocopherol had a lower risk of cataract than those with a lower serum tocopherol [75] and lens opacities were associated with lower levels of riboflavin, vitamin E, Fe, and protein nutritional status [76]. By contrast, McNeil et al. [77] found that vitamin E supplemented for 4 years at a dose of 500 IU daily did not reduce the risk of nuclear, cortical, or posterior subcapsular.
Carotenoids
The best studied carotenoids in the eye are lutein and zeaxanthin. Carotenoids are made up of more than 600 structural variants that are all lipophilic pigments. They are synthesized in plants, fungi, bacteria, and algae and provided to mammalians only in their diet, particularly in egg yolk and dark leafy green vegetables such as spinach or kale. Some carotenoids, including β-carotene, can protect against photooxidative damage by scavenging 1O2 and peroxyl radicals and can interact synergistically with other antioxidants [78]. They may lower the risk for several degenerative disorders, cardiovascular and ophthalmological diseases, and various types of cancer [79]. In the eye, lutein and zeaxanthin have been shown to filter high-energy wavelengths of blue visible light, primarily in the macula of the retina and to act as antioxidants that protect against the formation of ROS [3]. A study by Gale et al. [80] showed that the risk of nuclear cataract was lowest in people with the highest plasma concentrations of α- or β-carotene while the risk of cortical cataract was lowest in people with the highest plasma concentrations of lycopene. In addition the risk of posterior subcapsular cataract was lowest in those with higher concentrations of lutein but high plasma concentrations of vitamin C, vitamin E, or zeaxanthin and β-cryptoxanthin were not associated with decreased risk. In the Blue Mountain Eye Study, as mentioned above, an increased intake of vitamins C and E, β-carotene, and zinc was associated with a reduced risk of nuclear cataract but not with cortical or posterior subcapsular cataract [65]. The Beaver Dam Eye Study, also mentioned above, found that patients with the highest quintile of lutein intake were half as likely to develop nuclear cataract as those in the lowest quintile of intake [81]. In the Pathologies Oculaires Liées à l’Age (POLA) Study, the highest quintile of plasma zeaxanthin was significantly associated with reduced risk of nuclear or other cataract, among other carotenoids, only β-carotene showed a significant negative association with nuclear cataract [82]. In the Carotenoids in the Age Related Eye Disease Study (CAREDs), women in the group with high dietary levels of lutein and zeaxanthin had a 23 % lower prevalence of nuclear cataract compared with those with low levels [83]. Despite the conclusions of these studies, an analysis of a number of supplementation studies involving carotenoids using the FDA evidence review system, found no evidence to support the fact that lutein or zeaxanthin can protect against cataract formation [84].
Antioxidant Enzymes of the Eye Lens
The Superoxide Dismutases
The antioxidant enzymes of the eye catalytically remove ROS, in some cases generating other reactive or unstable species but generally resulting in a less toxic environment. Mitochondrial superoxide is dismutated to H2O2 and O2 by the superoxide dismutases. Manganese superoxide dismutase (MnSOD, SOD 2) is present in the mitochondrial matrix while copper zinc superoxide dismutase (CuZnSOD, SOD 1) is localized to the intramembrane space of the mitochondria and in the cytosol [19]. In the absence of metal ions and thus the Fenton reaction, H2O2 produced in this reaction can then be detoxified by specialized enzymes including glutathione peroxidase (GSH-Px), the peroxiredoxins (Prxs), and catalase, described below. A recent study examined total SOD (TSOD), MnSOD, and CuZnSOD activities in lens epithelial cells derived from different types of cataract in patients having phacoemulsification cataract surgery. The highest level of all TSOD, MnSOD, and CuZnSOD activity was in patients 50 years or younger with the activity gradually declining with age. The level of TSOD activity increased in cortical cataract and the individual levels of CuZnSOD and MnSOD activities in lens epithelial cells were also higher in cortical cataracts [85]. MnSOD has been shown to protect human lens epithelial cells against oxidative stress using up- and down-regulation of the enzyme in lens epithelial cells [86]. The intrinsic apoptotic pathway was also significantly increased in MnSOD down-regulated lens epithelial cells compared to those with up-regulated MnSOD when exposed to superoxide [87]. CuZnSOD, which is not mitochondrial specific and makes up 90 % of total SOD [88], was shown to prevent H2O2-induced oxidative damage when overexpressed in whole rat lenses [89]. Treatment of human lens epithelial cells with a bolus of 17 β-estradiol, known to protect lens cells against oxidative stress by preserving the mitochondria, resulted in a significant rapid increase in the activity of MnSOD with no effect on levels of mRNA or protein [90].
Hydrogen Peroxide Detoxifiers
B.1 Catalase
Human catalase is normally found localized in the peroxisomes, where it functions to decompose H2O2 to H2O. One study investigated the effect of over expressing catalase in both nucleus and mitochondria of mice, two areas normally devoid of catalase activity. They found that oxidative damage was reduced, H2O2 production and H2O2-induced aconitase (an enzyme involved in the TCA cycle) inactivation were attenuated, and importantly that cataract development, cardiac pathology, and the development of mitochondrial deletions were reduced [91]. Resveratrol (a polyphenol antioxidant found in plants) was found to protect human lens epithelial cells against H2O2-induced oxidative stress by increasing catalase, SOD-1, and heme oxygenase (HO-1) expression [92]. In rat lens epithelial explants, increased levels of GSH and catalase suppressed changes typically associated with TGFβ-induced transdifferentiation including wrinkling of the lens capsule, cell-surface blebbing, apoptotic cell loss, induction of αSMA, and loss of Pax6 expression [93]. However, lenses from mice deficient in catalase did not show any increased susceptibility to oxidative stress generated by photochemical reaction [94], suggesting that catalase is not the most important enzyme involved in decomposing H2O2.
B.2 The peroxiredoxins
Given its peroxisomal location and the evidence outlined above it is unlikely that catalase is the major H2O2 detoxification enzyme in the lens. One likely H2O2 detoxification system in the lens is the Prxs, which are peroxide scavengers that possess redox active cysteines and use the thioredoxin system as an electron donor to detoxify H2O2, OONO− and a wide range of hydroperoxides [95]. Six mammalians Prxs are known, with Prx 1, 2, and 6 found in the cytoplasm, Prx 3 in the mitochondria, Prx 4 in the endoplasmic reticulum, and Prx 5 found in various compartments in the cell including the peroxisomes and mitochondria [95–97]. Since the mitochondria is the major source of H2O2 formation, Prx3 is well studied. Significant levels of Prx 3 mRNA and protein have been detected in human lens epithelial and fiber cells and it has been shown that Prx 3 is inducible by H2O2 in human lens epithelial cells but not by tBHP or heat shock [97].
B.3. Glutathione peroxidase
Another potentially important H2O2 detoxification enzyme is GSH-Px which also reduces H2O2 to H2O or alkyl peroxides to alcohols at the expense of reduced GSH [33]. In the lenses of H2O2-treated mice with deficient levels of GSH-Px, DNA strand breaks in the mice with elevated GSH-Px were 40 % of those with normal GSH-Px activity [98], while in the GSH-Px knockout mouse lens DNA damage was fivefold that of GSH-Px rich transgenic mice [98].
Free Metal Detoxifiers of the Eye Lens
Metallothioneins
Fenton-type reactions, where H2O2 reacts with free metal ions i.e., Iron (Fe2+) or copper (Cu2+) to produce the •OH radical, are a major source of oxidative stress initiated by transition metals [99] and are thought to be involved in the formation of cataract [100, 101]. Detoxification of these metals by metallothioneins in combination with careful control of free iron levels by ferritin is central to prevent ROS production. Human exposures to toxic metals such as Fe, Cu, cadmium (Cd), lead, aluminum, mercury and others may arise from fossil fuel emissions, industrial waste, cigarette smoke, and air pollution [102]. These metals have been associated with increased risk of cataract formation [103] and it has been proposed that detoxification of metals may play a major role in prevention of cataract. In support of this theory, increased Cd levels [104] and increased Cu levels [105] have been reported in cataract compared to clear lenses. Another study that compared corticonuclear and mature cataracts found higher Fe levels in the mature cataract [106] suggesting a role for these metals in cataract formation. Metallothioneins (MTs) serve to detoxify free metals; there are 16 known isoforms of MTs in humans, grouped into four classes: I, II, III, and IV. The human lens expresses MT classes I and II. Only one isoform, MTIIa, is specific for the lens epithelium, whereas the MTI isoforms are expressed at lower levels in both the lens epithelium and lens fibers [107]. In addition, MTIIa exhibits increased expression in age-related cataract compared with clear human lenses [108] suggesting a possible role for MTIIa in lens protection. Lens MTs (Ig, If, Ih, Ie, and IIa) were differentially induced by specific metals in human lens epithelial cells, specifically by Cd2+ and Zn2+, but not Cu2+ [109]. Similar responses of the MTIIa gene were detected in identically treated primary human lens epithelial cells. Cd2+ and Zn2+ induced MTIIa to five times higher levels than MTIg [109]. Overexpression of MTIIa in lens epithelial cells has been shown to protect against Cd as well as TBHP-induced oxidative stress [110]. The same study also shows that MTIIa may play a role in regulating expression of other important antioxidant genes, HO-1, thioredoxin reductase, and MnSOD, antioxidant molecules that could further enhance protection against oxidative stress in lens cells, potentially delaying onset of cataract [110].
Ferritin
Fe is transported in the plasma using transferrin, the Fe transport protein, while ferritin is the intracellular protein responsible for iron binding and storage. Ferritin, which is found throughout the lens [111, 112], is a multimeric iron-storage protein consisting of 24 subunits of two types: heavy (H) and light (L). The ratio of these two chains is tissue specific and controls iron storage and availability [113]. Fe levels in the eye lens have been reported to be between 0.18 and 9.6 μg/g wet weight [114]. A number of studies have found increased Fe levels in cataractous lenses [106, 111] with redox active Fe (not bound to ferritin) also found at higher levels in cataractous compared to control lenses [100, 101]. Low levels of Fe have been found in both the aqueous and vitreous humors [115]. Levels are kept low by the blood ocular barrier preventing transferrin entrance into the eye [114]. Inflammation which causes a breakdown of this barrier results in large increases in iron concentration in both the aqueous and vitreous humors [116]. The lens tightly controls Fe levels and during inflammation the lens accumulates Fe by taking it up from increased levels in the aqueous and vitreous humors in both the transferrin and non-transferrin-bound forms. The lens Fe concentration returns to control levels following resolution of the inflammatory episode [117]. Why the lens would act as a sink for excess Fe is not known and is the subject of much research. In a recent study using knockdown of ferritin heavy chains in human lens epithelial cells, increased iron availability resulted in increased cystine uptake and GSH concentration and decreased nuclear translocation of hypoxia-inducible factor 1-α (a transcription factor that regulates vascular endothelial growth factor (VEGF) expression) and VEGF accumulation in the cell-conditioned medium [118].
Protein Repair Systems of the Eye Lens
Methionine Sulfoxide Reductases
While all amino acids are susceptible to oxidation, methionine and cysteine residues are among the most vulnerable due to the presence of a sulfur containing side chain that is sensitive to attack from ROS such as H2O2, •OH, hypochlorous acid, chloramines, and OONO− [119, 120]. In the eye lens protein methionine sulfoxide (PMSO), the oxidized form of methionine, levels increase upon aging [121] and in human cataractous lenses 60–70 % of total lens protein is found as PMSO [122]. ROS-mediated oxidation of methionine results in an asymmetric sulfur center and thus PMSO exists as two epimers, the Sand R-epimers. Methionine oxidation in proteins can result in altered conformation, activity, sub-cellular localization patterns, and aggregation states which are associated with loss of cellular functions, apoptosis, and cell death [123]. Methionine oxidation is, however, reversible via a thioredoxin dependent reaction in which PMSO is converted to reduced methionine. This reaction is catalyzed by a family of enzymes called the methionine sulfoxide reductases (Msrs). The Msr family consists of MsrA (found in the cytosol and mitochondria) and three MsrBs; MsrB1 (localized in the cytosol and nucleus), MsrB2 (localized in the mitochondria), and MsrB3 (localized in the endoplasmic reticulum and mitochondria) that act on the S- and R-epimers respectively [124]. The oxidized thioredoxin produced during the reduction of PMSO is subsequently reduced by thioredoxin reductase (TrxR) in an NADPH dependent reaction. It has been shown that in bovine lenses 40 % of Msr activity is due to MsrB while the remaining is MsrA [125]. MsrA has been shown to play an important role in protection of lens cells against oxidative damage and it has been shown to be required for the maintenance of lens transparency in vivo [126–128]. Gene silencing of MsrA decreases the resistance of lens epithelial cells to H2O2-induced oxidative stress resulting in increased mitochondrial ROS levels in human lens cells [126] and loss of lens cell mitochondrial function [24]. Similarly gene silencing of MsrB1, B2, and B3 results in decreased resistance to tBHP-induced oxidative stress and increased cell death in human lens epithelial cells [126]. Deletion of the MsrA gene in mice leads to oxidative stress-induced cataract [127]. By contrast, overexpression of MsrA in human lens cells protects against oxidative stress and preserves mitochondrial function [126]. Recently, both cytochrome c (cyt c) [127] and α-crystallin/sHSP [128] have been identified as key targets of MsrA function in the lens. Both proteins are critical for lens function. Cyt c is essential for mitochondrial electron transfer and is a key initiator of apoptosis in mammalian cells [129]. α- crystallin/sHSP is a molecular chaperone that is essential for the maintenance of lens transparency whose deletion has been shown to result in cataract formation [130–133] and is discussed in more detail below.
Thioltransferases
Thioltransferase, known interchangeably as glutaredoxin, is a GSH-dependent cytosolic protein and a member of the thiol-disulfide oxidoreductase enzyme family, containing a conserved CXXC active site [134]. Two isoenzymes of thioltransferase are known: cytosolic TTase-1 (Grx1) and mitochondrial TTase-2 (Grx2) [134]. The TTases use reduced GSH in their reaction to reduce protein thiols (s-thiolation) preventing disulfide bond formation and protein aggregation [134]. TTase activity was found to be higher in the lens epithelial layer than in the rest of the lens [135]. In cultured lens epithelial cells TTase 1 levels and activity were shown to be up-regulated by treatment with H2O2. Depletion of GSH and subsequent treatment with H2O2 also increased levels of TTase in human lens epithelial cells [136]. Porcine lenses cultured in H2O2 over a 24-h period showed similar trends for TTase, with slow transient increases in TTase activity, mRNA transcript, and protein levels in response to low level of H2O2 and a more rapid response to higher levels of H2O2 [137]. In a separate study, treatment of cultured lens epithelial cells with 0.1 mM H2O2 over 3 h inactivated the key glycolytic enzyme glyceraldehyde-3-phosphate dehydrogenase (G-3PD) by more than 50 % over the first 15 min, this returned to 80 % of normal activity by 180 min. Interestingly, the inactivated cellular G-3PD in the cell extract could be partially reactivated by human recombinant TTase but not GSH [138]. The absence of TTase 1 was also shown to increase lens cell susceptibility to UV-B radiation (UVRB), light scattering was increased in TTase−/− mouse lenses compared to wild type mouse lens after treatment with UVRB [139]. TTase-2 has been shown to protect against disruption of the mitochondrial transmembrane potential in lens epithelial cells during oxidative stress conditions [140] and more recently TTase-2 was shown to protect complex I of the electron transport chain and to prevent H2O2-induced apoptosis in lens epithelial cells [141].
Reducing Systems of the Eye Lens
Thioredoxin and Thioredoxin Reductase
This section could easily contain GSH which we have grouped under ROS scavengers. It could also contain a section on NADPH, which is required for Msr repair of PMSO, thioredoxin repair of disulfide bonds and the recycling of GSH but NADPH is mentioned specifically in many other sections in this review. Thioredoxin (Trx) is the major disulfide reductase responsible for maintaining proteins in a reduced state within the cell. It is a small, cysteine rich protein possessing a dithiol/disulfide active site (CGPC) that acts to reduce protein disulfides. Its action results in an internal disulfide on the thioredoxin protein itself and this must be reduced by TrxR in an NADPH dependent reaction in order to recycle Trx. Trx serves as an electron donor, via the thiol/disulfide exchange reaction, for a number of enzymes including Msrs, ribonucleotide reductases, as well the peroxiredoxins [142]. Reduction of disulfide bonds is thought to be an important regulatory step where Trx could control the redox state of critical SH groups involved in structure and catalytic function in proteins. There are two nuclear encoded isoforms of Trx, Trx 1 found in the cytosol, and Trx 2 which is localized solely to the mitochondria. Trx 1 not only is an important part of the antioxidant defensive against oxidative stress but it also plays a role in transcription, growth control, and immune function [142]. Early embryonic lethality results from knockout of either Trx 1 [143] or Trx 2 [144] and it has also been shown in mice that a haploinsufficiency (a single functional copy of a gene, where insufficient product is made) of Trx 2 results in the reduction of ATP production and increased ROS production [145]. Early work on mouse lenses revealed that in vivo photochemical oxidative stress to Emory mice resulted in a fivefold up-regulation of the Trx 1 gene in the lens at 3 weeks and a fourfold increase of the lens Trx 1 protein but that Trx 2 was unchanged [146]. In cultured lens epithelial cells, Trx 1 is up-regulated at both transcript and protein levels in response to H2O2 treatment [147]. Interestingly, human lens epithelial cells treated with exogenous human recombinant Trx 1 showed a simultaneous increase in mRNA expressions of mitochondrial MnSOD, TTase 1, TTase 2, and thioredoxin peroxidase IV (Prx3) [148]. Recently a study on 23 normal human lenses of 19–77 years which were grouped into 2nd, 3rd, 5th, 6th, and 7th decades, indicated that Trx 1 activity decreased with age. Activity of Trx 1 in the 7th decade appeared to be 30 % of the activity found in lenses from the 2nd decade, a significant drop in activity. Interestingly the corresponding protein levels of Trx 1 were unaffected and remained steady throughout the seven decades in the lenses studied [149].
Thioredoxin-Like Proteins
Recently a thioredoxin-like protein (TXNL) containing the conserved CXXC reducing motif was discovered in the retina of mice and termed rod derived cone viability factor (RdCVF) [150]. This protein known as TXNL6 in humans is a product of the NXNL1 gene. The discovery of a novel CXXC containing protein led to the hypothesis that other reducing systems could function in the eye in addition to or as an alternative to the better characterized reducing systems such as Trx. TXNL6 is part of a small family of thioredoxin-like proteins which consists of TXNL1, TXNL2, TXNL4, and TXNL5. TXNLs share the CXXC active site of Trx 1 and/or the thioredoxin fold which consists of a four stranded β sheets surrounding three α helices [151]. Little is known about the disulphide reducing action of many of the TXNLs but recent work has shown that TXNL6 is present in both the lens epithelium and lens fiber cells, that it is inducible by oxidative stress and that it can serve as a reducing agent for MsrA in the repair of essential lens proteins [152]. TXNL1 is known to be a redox sensor and part of the 26 s proteasome subunit [153, 154]. TXNL2 is also known as glutaredoxin 3 and may catalyze the reduction of glutathione mixed disulfides [155]. Little is known about the oxidoreductase role of TXNL4 if any but it is known to be essential for the G2/M transition of the cell cycle [156]. TXNL5 is known to have redox potential similar to that of Trx 1 [157] and it is known that it can itself be reduced by TxrR and that it inhibits TNF-α induced NFκB activation to a greater extent than Trx 1 [158].
Chaperone Proteins of the Eye Lens
α-Crystallin
Heat shock proteins (Hsps) are highly conserved proteins that are induced in response to various physiological and environmental stressors [159]; interestingly HSPs form essential partnerships with the proteasome and lysosomes in protein degradation processes [159]. The molecular chaperones expressed in lens epithelial cells include αA-crystallin, αB-crystallin, Hsp25/27, Hsp40, Hsc70, Hsp70, and TCP-1 [160–163]. In the lens the predominant Hsp is α-crystallin. α-crystallin is made up of two small HSPs (sHSPs); αA and αB-crystallin, α-crystallin makes up approximately 40–50 % of total lens protein and is crucial for maintaining lens transparency. In addition to its structural and refractive role in the lens, α-crystallin is a molecular chaperone [130, 132] that functions to prevent protein aggregation [131], and formation of high molecular weight aggregates in the lens. α-crystallin has also been implicated in apoptotic control and cell survival [9]. In its native state in the lens, α crystalline consists of two subunits called αA- and αB-crystallin that consist of 173 and 175 amino acids respectively. αA- and αB-crystallin share as much as 57 % homology and exist in the lens in a 3:1 ratio [164]. Deletion of αA-crystallin in mice results in smaller lenses compared to wild type, and the development of opacification that spreads with age [133]. Mutation of αA-crystallin (R116C), which causes loss of chaperone function, leads to cataract in humans [165]. Similarly a mutation of αB-crystallin (R120G) that causes cataract and desmin-related myopathy in humans, also leads to loss of chaperone function [166]. Importantly, the chaperone function of α-crystallin is also lost upon oxidative conditions [128, 167, 168]. Specific oxidation of bovine α-crystallin methionines to PMSO resulted in loss of chaperone function in vitro [128] and αA-crystallin methionines are found as PMSO in rat hereditary cataracts [169] and in MsrA knockout mice [128]. Oxidation by Fenton-type reaction of rat recombinant αA- and αB-crystallin was shown to result in higher molecular weight proteins that lacked chaperone function [170]. These results suggest that oxidation of α-crystallin results in loss of chaperone function and that loss of chaperone function plays a role in cataract formation. αA-crystallin and αB-crystallin as well as HSP27 can be induced by specific metals in SRA01/04 human lens epithelial cells. Cd2+ and Cu2+, but not Zn2+, induced αB-crystallin and HSP27 while αA-crystallin was induced by Cu2+ only [109].
References
1.
Michael R, Bron AJ. The ageing lens and cataract: a model of normal and pathological ageing. Philos Trans R Soc Lond B Biol Sci. 2011;366(1568):1278–92.PubMedCentralPubMed
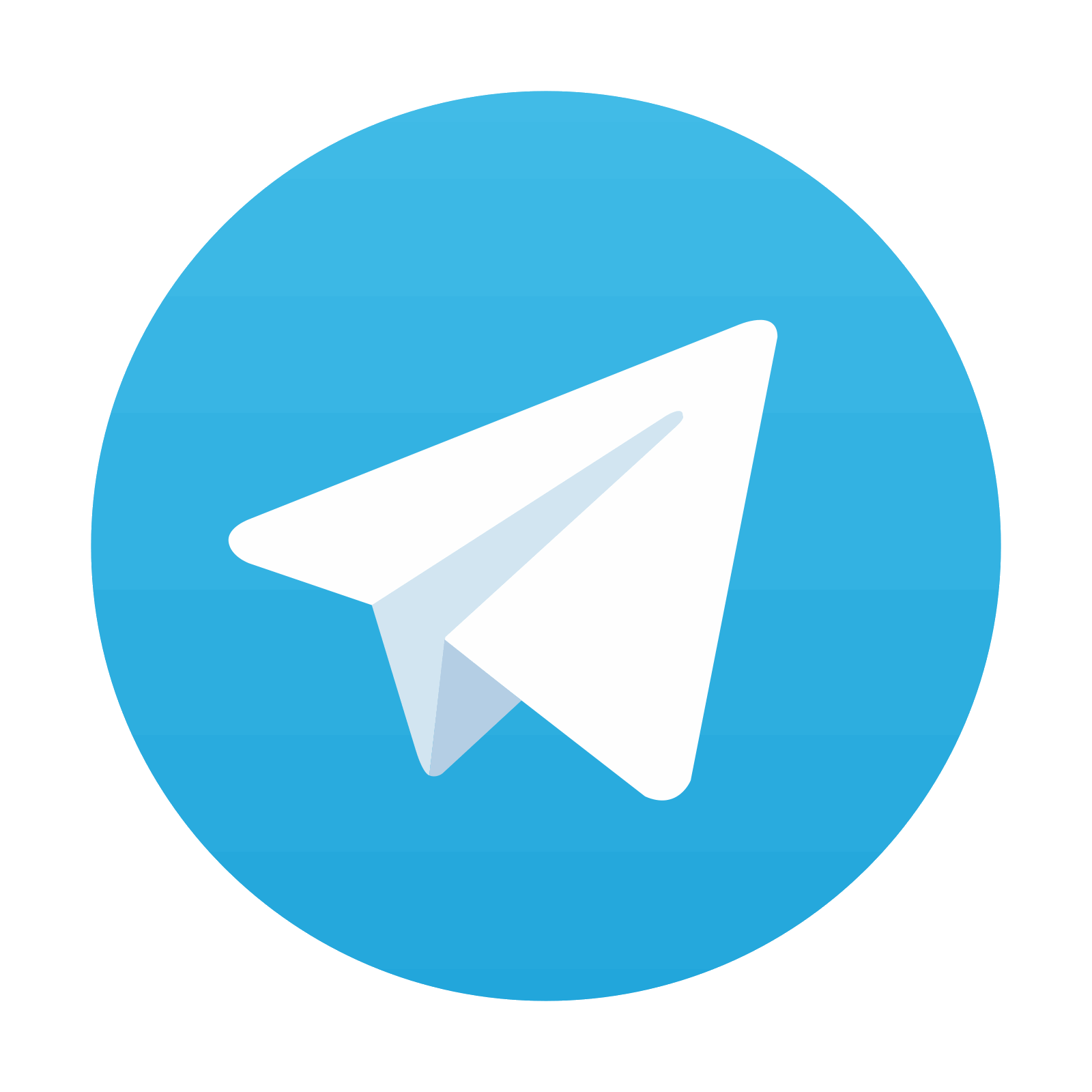
Stay updated, free articles. Join our Telegram channel
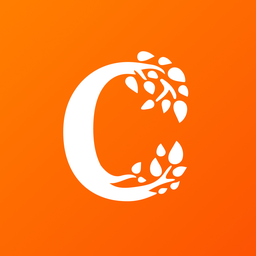
Full access? Get Clinical Tree
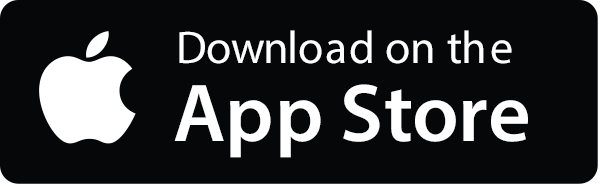
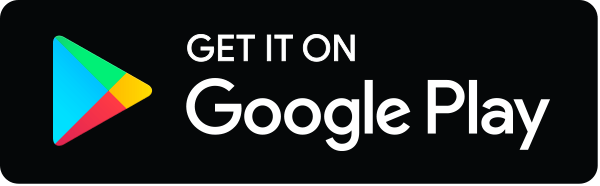