Fig. 64.1
OCT imaging of a bioreactor with its engineered tissue construct. (a) Schematic of the bioreactor. (b) Transverse and (c) longitudinal OCT scan. The bright pixels within the construct represent the cells. The outer interface of the bioreactor in OCT image is not shown (Reprinted with permission from [31])
64.3.3 OCT Imaging of Scaffold and Substrate
Scaffolds used in tissue engineering act as templates and provide mechanical, chemical, and biological support for tissue regeneration. They are commonly 3-D structures with porous or fibrous degradable structures. The parameters of the scaffold, such as material, pore size, porosity, and pore interconnection, affect the cell activity and cell distribution within the scaffolds. OCT can evaluate and monitor the architecture of the scaffolds and the cellular distribution without sample preparation, which provides instant feedback on the growth and development of the engineered tissue.
Recently, OCT along with appropriate imaging processing algorithms was used to investigate parameters of the porous scaffold, including volume porosity, pore interconnectivity, and pore size [54]. Accurate characterization of these parameters provides valuable feedback for better control of the fabrication conditions. Figure 64.2 shows OCT images of porous hydrogel scaffolds fabricated using the porogen-leaching technique [55] with different formulations. The images are obtained using a swept-source Fourier-domain OCT system (Thorlabs Inc.) with a laser source at 1,300 nm and a broadband spectrum of 100 nm, which provided an axial resolution of 8 μm. The micropores and hydrogels are well resolved in both the cross-sectional and en face images, which also reveal the pore size and density. The color-coded 3-D-labeling images show the interconnectivity of different groups of pores. Based on this information, and assisted with appropriate algorithms, quantitative pore density and interconnectivity can be determined [54].
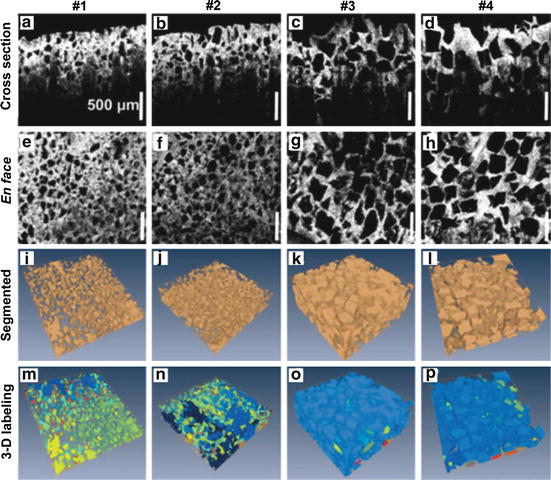
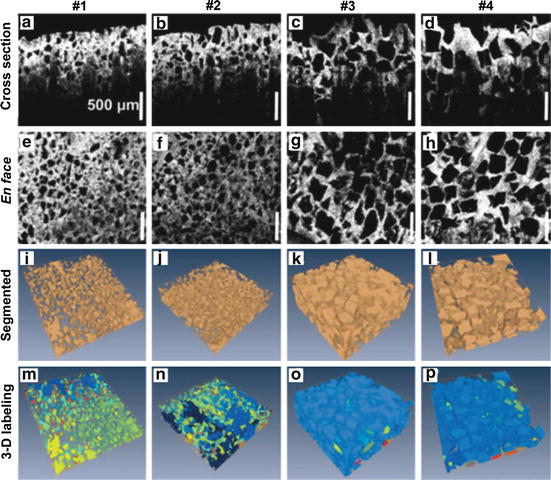
Fig. 64.2
(a–d) Cross-sectional and (e–h) en face OCT of scaffolds with different volume porosities. For scaffolds #1–4, the calculated volume porosities are 24.4 % ± 7.5 %, 18.1 % ± 10.3 %, 41.4 % ± 6.0 %, and 39.7 % ± 7.7 %, respectively. En face images are taken at a depth of 100 μm. (i–l) 3-D observation of the pores segmented from OCT images. (m–p) 3-D observation of isolated and connected pore groups through the 3-D labeling process. Different colors indicate different groups. 3-D images are analyzed using a depth range of 0–300 μm (Reprinted with permission from [54])
In order to provide a better nutrient supply to the porous and thick 3-D constructs, the integration of a perfusion method becomes essential in various bioreactors. In addition to a better exchange of nutrients and waste, perfusion generally exerts mechanical stimulation on the engineered tissue, which accelerates the maturation of the constructs. It is essential to attain the information about the relationship between perfusion rate and fluid shear stress in 3-D porous scaffolds and their dependence on the main parameters of the scaffolds such as pore density, pore size, and shape. Potentially, this can be done with DOCT (Doppler optical coherence tomography), a functional variant of traditional OCT. DOCT uses additional phase information from the complex OCT signals, which can be used to monitor the directions and velocities of moving particles in the backscattered spectrum. It is therefore possible to provide information regarding both the scaffold architecture and local fluid flow through 3-D scaffold, simultaneously.
The shear stress from fluid flow can be directly related to the fluid velocity measured by DOCT:
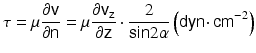
where V is the flow velocity vector and V z is the component of the velocity in the z direction (probe beam direction) measured by DOCT. This calculation and other technical details regarding DOCT can be found in ref. [56] and Chaps. 42, “Doppler Fourier Domain Optical Coherence Tomography for Label-Free Tissue Angiography” and 45, “Optical Coherence Tomography in Cancer Imaging” in this book. In addition, since DOCT can provide both the 3-D microstructural morphology and 3-D flow map for each pores in a scaffold, the combination of the structural and flow images permits the direct measurement of porosity and interconnectivity for the porous scaffolds. The porosity is defined as the ratio of total pore volume to total scaffold volume as determined by the OCT structural images, which is typically determined by block image processing.
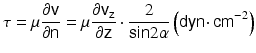
(64.1)
A typical cross-sectional OCT image of a low-porosity chitosan scaffold (LPCS) with a pore size ranging from 30 to 100 μm is presented in Fig. 64.3a. The chitosan scaffold is fabricated by a freeze-drying technique using a 2 % chitosan solution in acetic acid. The scaffolds, with different porosity and pore size, were obtained by adjusting the freezing rate [58]. A spectral-domain DOCT system, which used a superluminescent diode with a central wavelength of 842 nm and a measured axial resolution of ∼7 μm in air, was used to acquire these images. The bidirectional flow velocity map obtained by DOCT is presented in Fig. 64.3b. Both the distributions and the magnitudes of the flow can be seen. The magnitudes of bidirectional flow velocities were calculated and plotted in Fig. 64.3c. It is seen that there are variations of velocities within pores, indicating a heterogeneous distribution of flow velocity in the porous structures. Although the input flow rate was constant, the local fluid flow in this complex construct varied greatly in both magnitude and direction. Furthermore, the flow in the micropores did not show parabolic distributions. Consequently, the fluid shear stress, as shown in Fig. 64.3d, differed from pore to pore, with values ranging from 0 to 1.65 dyn cm−2. Nevertheless, the maximum fluid shear stress was generally located at the pore walls. Based on the quantitative comparison between the distributions of shear stresses at the pore walls of scaffolds with different porosity, dependence of shear stress on porosity, pore interconnectivity, and shape can also be characterized.
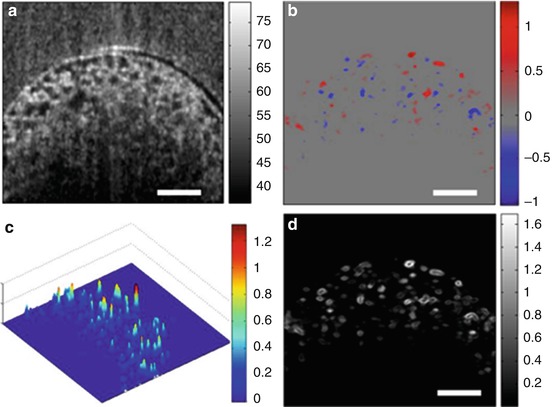
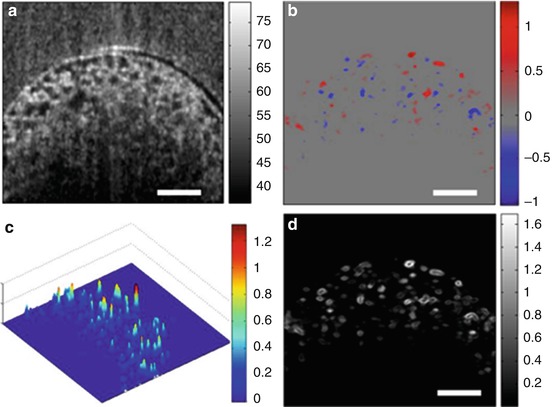
Fig. 64.3
DOCT monitored the fluid flow in a low-porosity chitosan scaffold in situ. Shown are, respectively, (a) the microstructural image, (b) the bidirectional local fluid flow map, (c) the 3-D plot of magnitudes of the flow velocities, and (d) the corresponding local shear stress distribution in a typical X-Z section. The units for color bars shown are (a) dB, (b) mm/s, (c) mm/s, and (d) dyn/cm2 respectively. The white scale bars indicate 200 μm (Reprinted with permission from [57])
The real flow rate can be further investigated with the technology called as Doppler optical microangiography (DOMAG) [59]. DOMAG combines the phase-resolved technique in DOCT and flow signals from optical microangiography (OMAG), which generates low-noise flow images, reflecting the real flow rate more precisely. Comparison between OMAG and DOCT on the fluid flow measurement in chitosan scaffolds (with round-shaped pore structure) is shown in Fig. 64.4. Figure 64.4a is the structural image of the scaffold. Figure 64.4b, c are from DOCT and Fig. 64.4d, e are from DOMAG for flow rate (d) and shear stress (e), respectively. There are two improvements for these images. First is the higher penetration depth of the image and second is the much clearer flow pattern in each pore using the DOMAG technique, which resulted in more accurate shear stress images (Fig. 64.4e). It is reported that the background noise from a non-flow region of the scaffold and random noise were imposed onto DOCT flow images, resulting in a difficulty to precisely measure small flow velocity [57, 59]. DOMAG is able to tackle this issue and separate the background flow signals from the background signals, which leads to low-noise images.
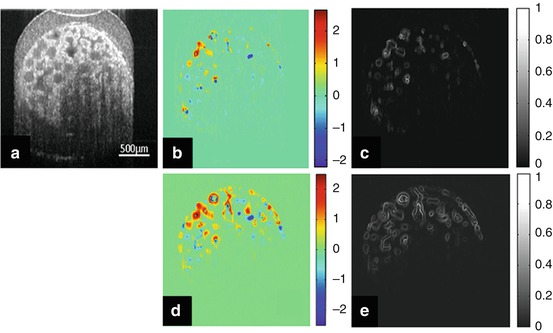
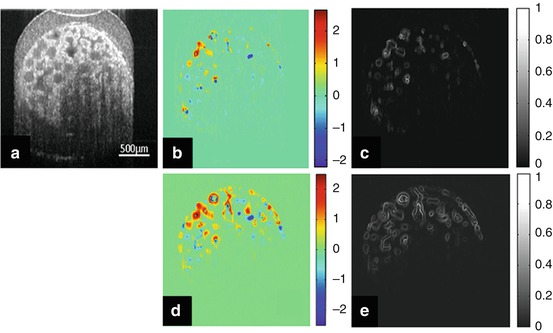
Fig. 64.4
(a–c) DOCT images for chitosan scaffolds with round-shaped pore structures: (a) structural image, (b) flow rate, and (c) shear stress. (d–e) DOMAG images of the same scaffolds in (a), showing the better resolution of the flow rate (d) and shear stress (e)
In addition, the mechanical properties are important parameters of a scaffold, which affect the development and regeneration of the tissue [60, 61]. The relationship between cell dynamics and the mechanical properties of a scaffold is complex. Cell activities are affected by the mechanical parameters of the environment, but these mechanical properties also have considerable impact on the growth and the behavior of cells. A good understanding and control of this interaction will help us improve regeneration of the desired tissue. Normally, the biomechanical properties of tissues are characterized by elastography. Traditionally, this has been performed with ultrasound or MRI, but recently elastography has been demonstrated in OCT in a technique called optical coherence elastography (OCE). One initial application has been for investigating the biomechanical properties of myocardial tissues [62]. The high resolution and sensitivity of OCE makes it suitable for detecting changes at the cellular level in engineered tissues [62]. As cells seeded in scaffolds proliferate and the engineered tissue develops, the cells increase in number and secrete extracellular matrix. During this process, the biomechanical properties of the engineered tissue will be altered, and these subtle changes can be detected with OCE in a spatially and temporally resolved manner.
Figure 64.5 shows a representative array of structural and OCE images acquired from a 3-D scaffold, with half of the scaffold seeded with cells and the other half serving as a cell-free control. The samples were imaged over a culture time of 10 days, and at each time point, the OCE images were analyzed to generate both displacement and strain maps, which are color coded to indicate regions of higher stiffness. The cell-seeded regions increased significantly in stiffness over the culture period, demonstrating that OCE could be used to measure the stiffness and biomechanical parameters from the engineered tissue in a spatially resolved, nondestructive manner. Related to engineered tissues, OCE was used to image the changing biomechanical properties in the developing Xenopus laevis tadpole, which exhibited a more markedly different range of tissue stiffness attributed to organo- and morphogenesis [63]. Much of our understanding of the complex processes in the field of developmental biology (Chap. 67, “Optical Coherence Tomography for Gastrointestinal Endoscopy”) can be applied to the field of tissue engineering, where we wish to control the growth and organization of engineered tissues in 3-D and under an array of pharmacological and mechanical stimuli.
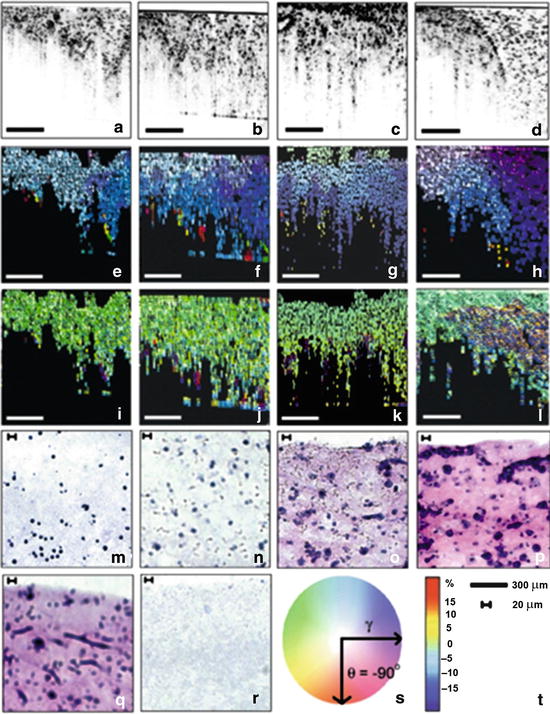
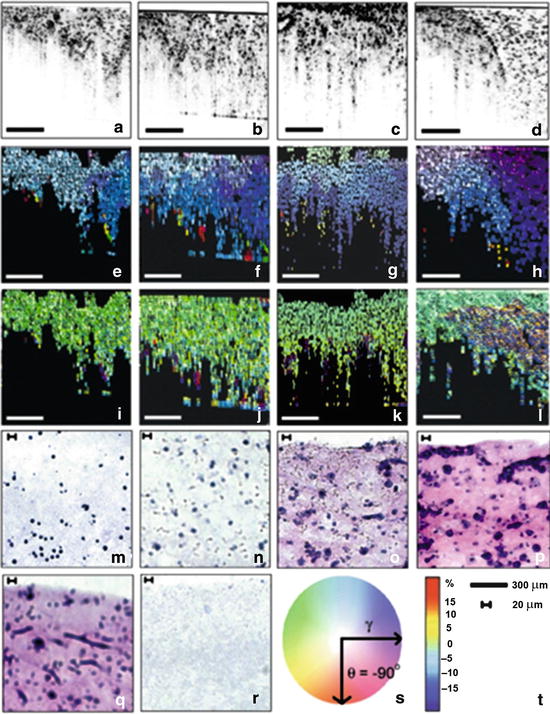
Fig. 64.5
Optical coherence elastography of engineered tissues. (a–d) Structural OCT images on day 0, 3, 7, and 10, respectively, of the boundary between the cell-seeded region (left) and the cell-free region (right). (e–h) Displacement maps on day 0, 3, 7, and 10, respectively, color coded using the scale in (s). (i–l) Strain maps on day 0, 3, 7, and 10, respectively, using the color scale in (t). (m–p) Corresponding histology from the cell-seeded tissue regions. (q) Histological image of cells after 10 days of incubation without embedded microspheres. (r) Histological image of a cell-free scaffold and microspheres. The size scale bar represents 300 μm in (a–l) and 20 μm in (m–r) (Reprinted with permission from [63])
64.3.4 OCT Imaging of Cell Dynamics
64.3.4.1 Cell Growth Profiles
In addition to bioreactors and scaffolds, activities of cells in these environments eventually determine the regeneration of the tissue. Because the environmental parameters have a critical influence on cell growth and the deposition of matrix, revealing the cell growth profiles within scaffolds will greatly increase our understanding of how to control culture conditions and optimize growth patterns. OCT has been utilized in this regard [54, 64–68]. Because of the difference in refractive index between cells and scaffolds, which results in the variation of backscattering of light from the different regions, OCT has the appropriate capability to investigate cell dynamics in engineered tissue.
Figure 64.6 shows OCT images, corresponding histology, and 3-D visualization of a fibroblast cell population seeded in a chitosan scaffold and imaged over several days, revealing the cell growth profiles and dynamics. Cells were cultured and imaged at days 1, 3, 5, 7, and 9. Initially, the chitosan scaffold provided very little scattering for OCT, as the index of refraction was similar to the surrounding media. As the seeded fibroblasts populated the scaffold, attached, and deposited extracellular matrix, the more highly backscattering extracellular matrix provided clear OCT signals, and OCT images revealed the porous structures of the scaffold. Interestingly, over time, the initial population of cells that were evenly distributed began to migrate toward the surface of the scaffold, likely in response to limited nutrients and waste exchange at the deeper regions. After 9 days, a high-density cell layer is observed at the surface and confirmed both with 3-D OCT and histological observations [64].
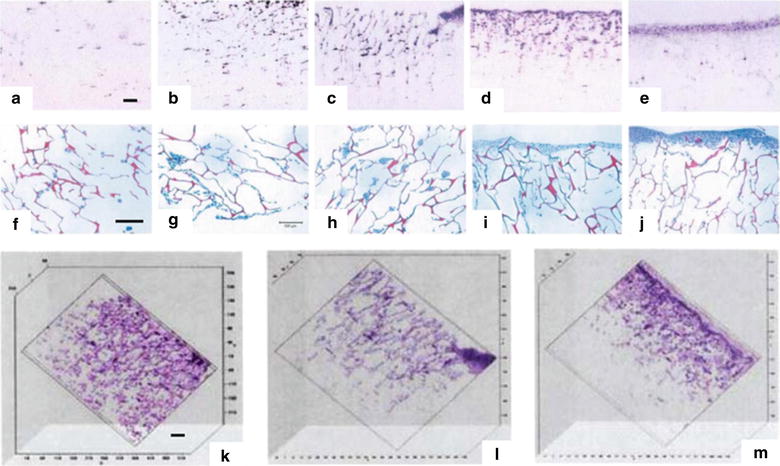
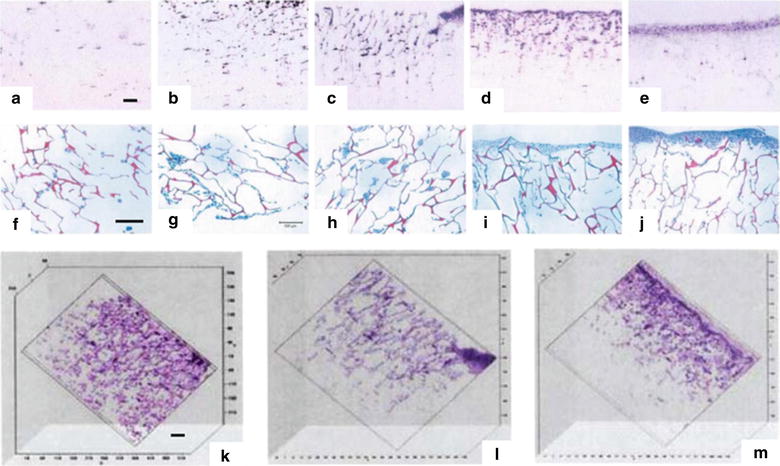
Fig. 64.6
Two- and three-dimensional OCT of a cell-seeded porous scaffold. Fibroblast cells were seeded in a chitosan scaffold and imaged at days 1, 3, 5, 7, and 9 (a–e, respectively) to show cell distribution changes. Initial cell seeding distribution is relatively uniform, but at later days, cells migrated toward surface to form a dense cell layer. Corresponding validating histology (f–j) is shown, along with 3-D OCT images at days 3, 5, and 7 (k–m, respectively). Scale bar represents 100 μm (Modified figure reprinted with permission from [64])
A quantitative measurement of cell growth is shown in Fig. 64.7. An OCT image from a blank poly (lactide) (PLA) scaffold immersed in water is shown in Fig. 64.7a. The scaffold has a 90 % porosity and 250–350 μm pore size. It can be seen that the pore structure is clearly delineated. An imaging depth of around 1.2 mm is achieved, despite the highly scattering nature of the scaffold. The imaging contrast is primarily provided by the difference in refractive indices between the polymer and water. The polymer wall reflects the light to a greater extent, thus appears brighter, while the pore appears as a darker area in the OCT image. Such contrast provides an opportunity to estimate the porosity from the OCT images. Figure 64.7b illustrates the OCT image acquired from a PLA scaffold seeded with MG63 bone cells and cultured for 4 weeks under static conditions. Structural changes are noted as compared to the blank scaffold. The pore size and porosity have decreased significantly, i.e., the darker areas in the images are reduced. From the cell seeding procedure and the expected cell growth profile, the increased brighter areas in the scaffolds are attributed to the cells and the extracellular matrix generated by the cells, increasing the optical scattering properties of the constructs and leading to an increased backscattering of the OCT signals.
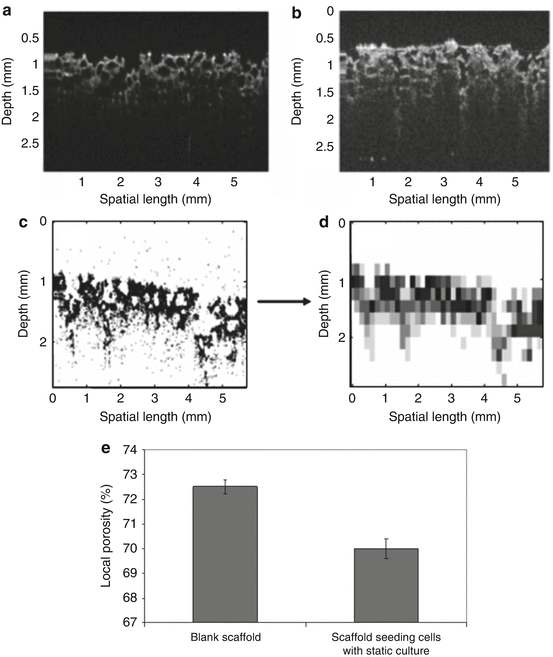
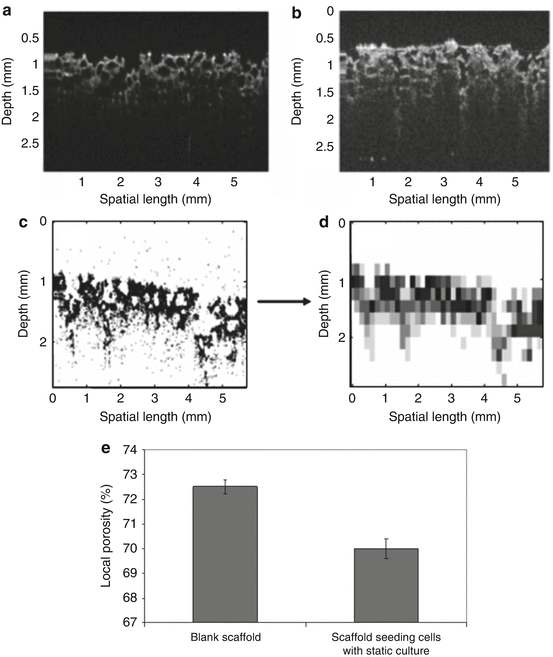
Fig. 64.7
OCT images porous scaffold seeded with cells. (a) A blank PLA scaffold and (b) a PLA scaffold seeded with MG63 bone cells following static culture for 4 weeks. (c, d) Accompanying diagrams illustrate an algorithm for calculating local porosity. (e) Resulting bar-chart comparison between a blank and seeded scaffold. The error bars represent standard deviation (Modified figures reprinted with permission from [56])
The pores within the scaffolds were initially filled with seeded cells. Occupation of pores continued as cells proliferated and differentiated, producing extracellular matrix. Thus, pore size and porosity of scaffolds become dynamic parameters in the culture period, which are closely correlated to cell growth profiles and tissue turnover. It is hypothesized that the quantification of porosity change with culture time and conditions can reveal information about the cell growth profile. A local porosity analysis of scaffolds has been proposed to quantify the porosity change, in which large and continuous defects in scaffolds (i.e., pore size >500 μm) are excluded. For each OCT scan, a threshold is set to discriminate between true empty pores and the pore walls. After binarization, the local porosity is calculated by a block processing method [56] demonstrated in Fig. 64.7c, d. The difference of porosity with statistical significance between a blank scaffold and cell-seeded scaffold is presented by this calculation method (Fig. 64.7e).
64.3.4.2 3-D Cellular Dynamics (Proliferation and Migration)
Our understanding of cell dynamics in 3-D engineered tissues has been limited by our ability to visualize these processes. The use of OCT may play a significant role in tracking these dynamic cellular processes in not only engineered tissues but also for a more basic understanding of fundamental cell behavior. The proliferation patterns of cells within the engineered tissue scaffolds can be monitored, as shown in Fig. 64.8. Three-dimensional OCT imaging shows expanding scattering regions of cells after several days in culture. The increase in scattering is likely the result of both increasing cell numbers in these focal regions but also from the deposition of extracellular matrix secreted by the viable cells.
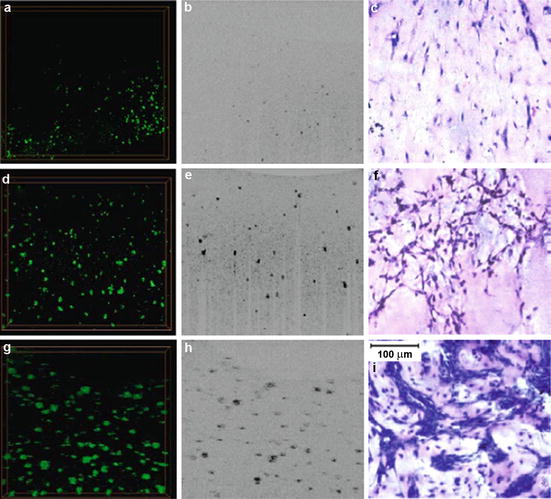
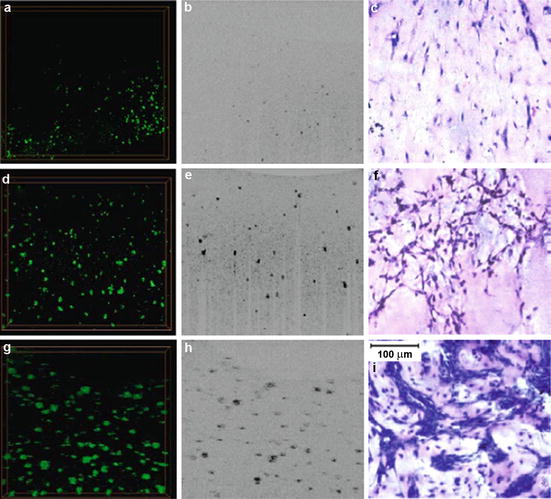
Fig. 64.8
OCT of cell proliferation in 3-D scaffolds. Images were acquired with low (5 × 104 cells) initial cell density. 3-D OCT images (a, d, g) and 2-D (x–z) OCT images (b, e, h) of engineered tissues were acquired on day 0 (a–c), day 4 (d–f), and day 8 (g–i). Histological images stained with H&E (c, f, i) are shown to confirm cell proliferation over time. Scale bar in (i) is applicable for all images (Figures reprinted with permission from [69])
In addition to cell proliferation, cell migration plays an important role in tissue growth and development. Using repeated OCT imaging over several hours, the migration of macrophage cells was tracked as they moved through a 3-D agarose scaffold in response to a chemoattractant gradient. Images in Fig. 64.9 show the color-coded 3-D spatial position changes over time, with the downward cell migration toward the chemoattractant noted.
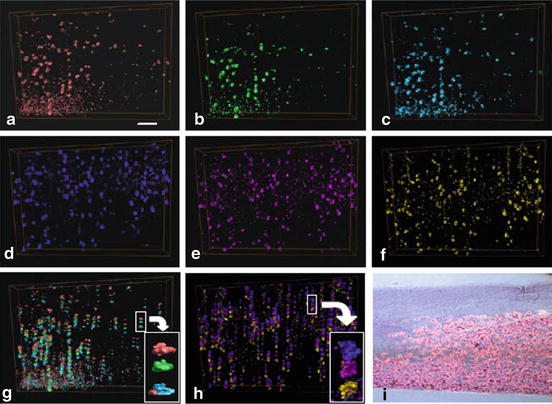
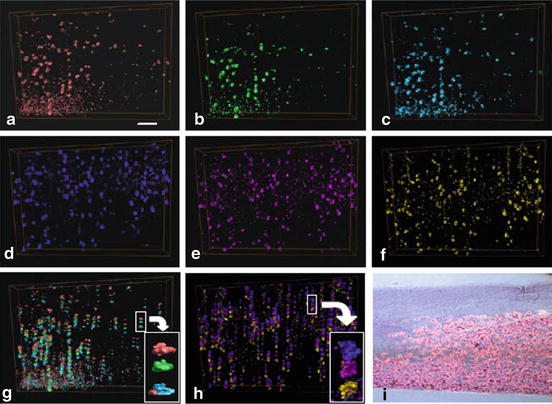
Fig. 64.9
OCT of cell migration. 3-D OCT images demonstrate the migration of macrophages (a–f). Cells at different points in time are labeled with different colors. The interval time is 40 min between (a/b, b/c, d/e, and e/f) and 120 min between (c/d). Individual OCT images are merged to form composite images of individual cell migration in 3-D space (g, h). Composite (g) is composed of (a–c) and composite (h) is a composed of (d–f). Insets in (g, h) show color-coded single-cell migration. Corresponding histology after the study is shown in (i), with macrophages collecting at the bottom. Scale bar is 200 μm (Reprinted image with permission from [69])
A second example of cell migration involves cell invasion through tissues, which is an essential step for tumor cells to metastasize. The invasive behavior of tumor cells in part depends on the cellular factors such as enzymes that enable cells to degrade their matrix components as well as on the characteristics of the extracellular matrix surrounding the cell. Therefore, a good knowledge of the cell-matrix interaction is useful when seeking treatment strategies aimed not only at cell killing but also at inhibition of tumor cell invasion and metastases. OCT offers a potential tool to observe and evaluate this process (Fig. 64.10). A model system with lung tumor cells grown on a collagen gel has been reported as a means to evaluate the tumor cell invasion ability [70]. The lung tumor cells grown on the gel with varying combinations of cell seeding number and collagen gel concentration have been scanned in OCT. It was found that OCT can differentiate cells and the gel. With 1 × 106 lung cancer cells seeding on a collagen gel of 2.5 mg/ml concentration, a well-defined two-layer OCT image was obtained: a thin, brighter layer on top of a homogeneous, thick, and darker layer (Fig. 64.10a). The boundary between the layers is sharply delineated. By the nature of the cell seeding procedure, the brighter top layer is ascribed to the cells, while the underlying layer corresponds to the gel. The well-defined two-layer image indicates that there is no cell migration into the gel. However, OCT of the tumor cells seeded on a collagen gel of 1.5 mg/ml concentration invading into the collagen gel can also be visualized and confirmed by histological section images. The OCT images of the tumor cells on the gel reveal invasion, as shown in the image as a brighter diffuse top cellular layer plus additional similarly bright cellular foci infiltrating within the gel layer (Fig. 64.10c). Validation by histological sectioning of the cell and gel complex has drawn the same conclusion, which demonstrates that OCT can become a convenient optical imaging tool to study the dynamic process of tumor cell invasion nondestructively and in real time.
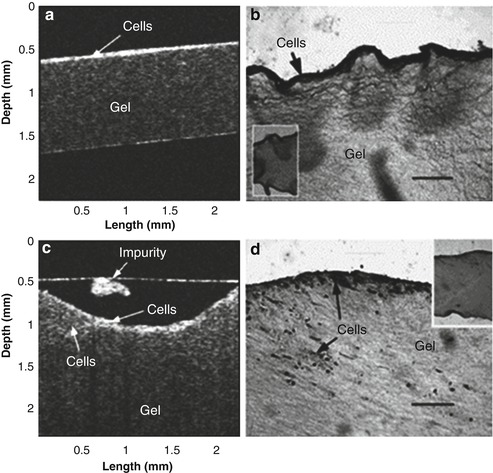
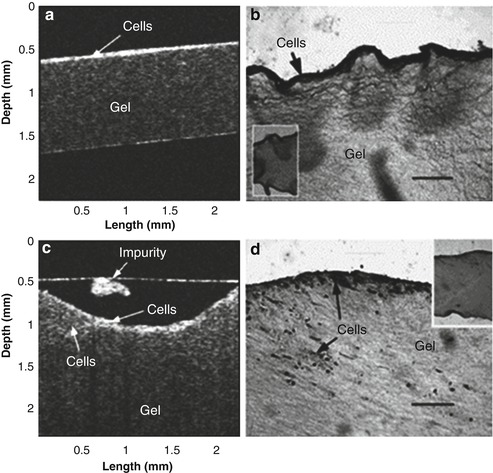
Fig. 64.10
OCT of tumor cell migration. (a) OCT image of lung cancer cells seeded on a collagen gel with (b) corresponding histological section of the specimen. (c) OCT image and (d) corresponding histology showing tumor cell invasion into the gel. Scale bar represents 100 μm (Reprinted with permission from [70])
64.3.4.3 Single Cell Identification and Differentiation
We have shown that OCT has the capability to image and monitor cell dynamics in engineered tissue, but only populations or clusters of aggregated cells are resolved. Higher imaging resolutions are needed to visualize single cells and subcellular organelles with OCT. The high-resolution variant of OCT is called optical coherence microscopy (OCM) which uses high NA optics and ultrabroad bandwidth light sources, such as a tungsten lamp, to realize high resolution in 3-D [40], where the axial resolution is governed by the bandwidth of light source, as in conventional OCT, and the transverse resolution is determined by the high NA optics. In contrast to the cross-sectional images of traditional OCT, OCM usually generates images of en face planes, as in confocal or multiphoton microscopy. OCM operates in two modes, i.e., full-field OCM (ffOCM) and scanning OCM. ffOCM has been used to visualize individual pores and cell populations in tissue-engineered constructs. Figure 64.11 provides an example of images acquired with ffOCM where bone cells (1 × 106) were seeded in a PLLA scaffold for 1 week, together with an image from a blank scaffold for comparison [67]. Here several clusters of cells with roughly spherical cell morphology are seen attached to the PLLA scaffold wall in the 3-D microenvironment. Although still in a developmental stage, it is likely that ffOCT could not only offer the ability to visualize small cell populations in 3-D microenvironment as well as their attachment to and interaction with the scaffold wall but also provide informative sensory feedback to optimize scaffold manufacturing.
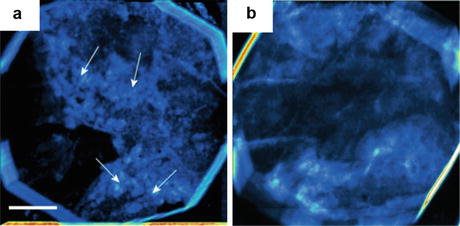
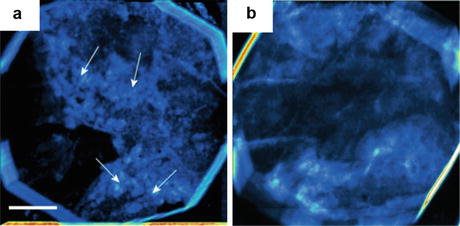
Fig. 64.11
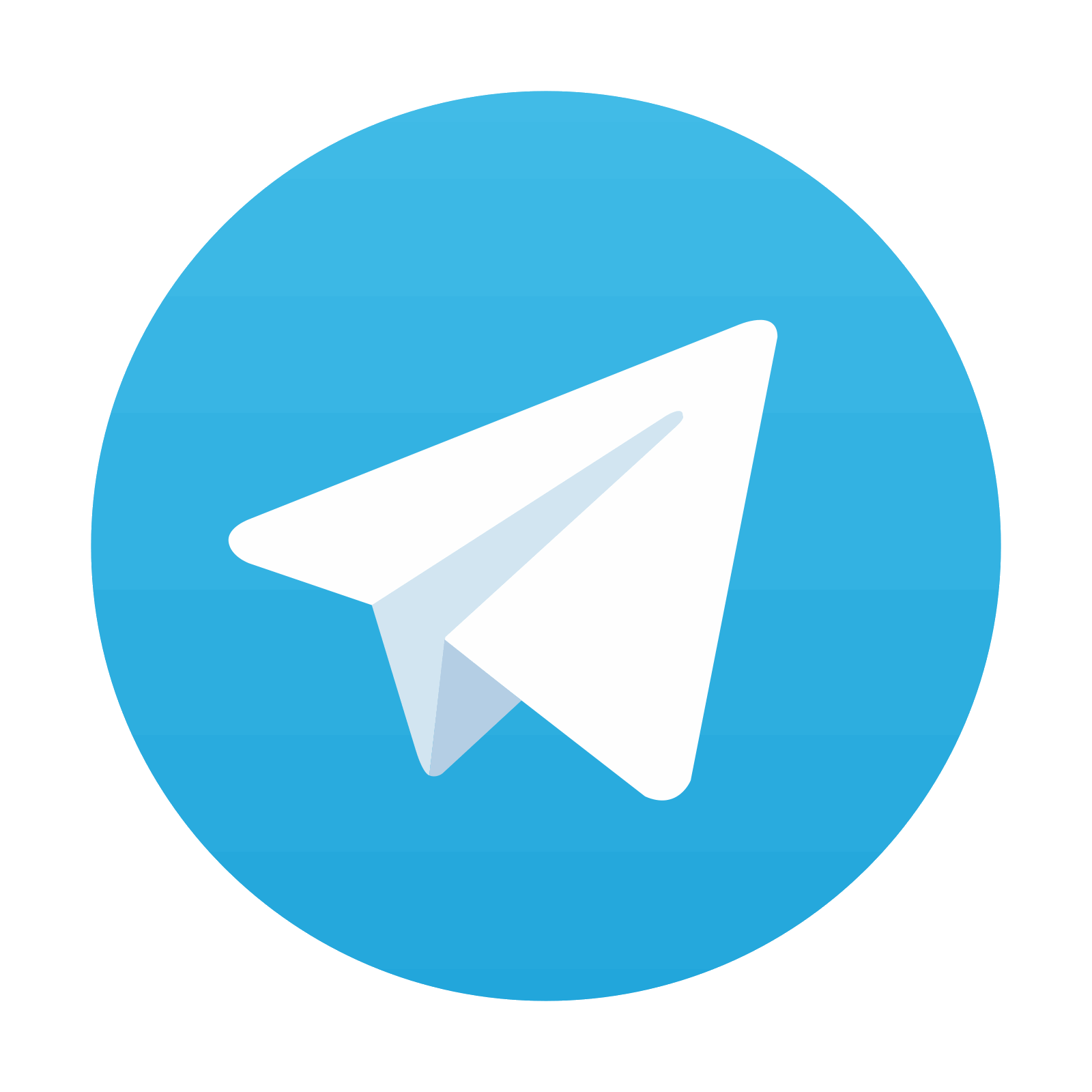
Full-field OCT images of a PLLA scaffold. (a) Scaffold seeded with 1 × 106 bone cells and cultured for 1 week, compared with (b) that of a blank scaffold. Representative examples of cells are indicated by arrows. Scale bar represents 50 μm (Modified figures reprinted with permission from [67])
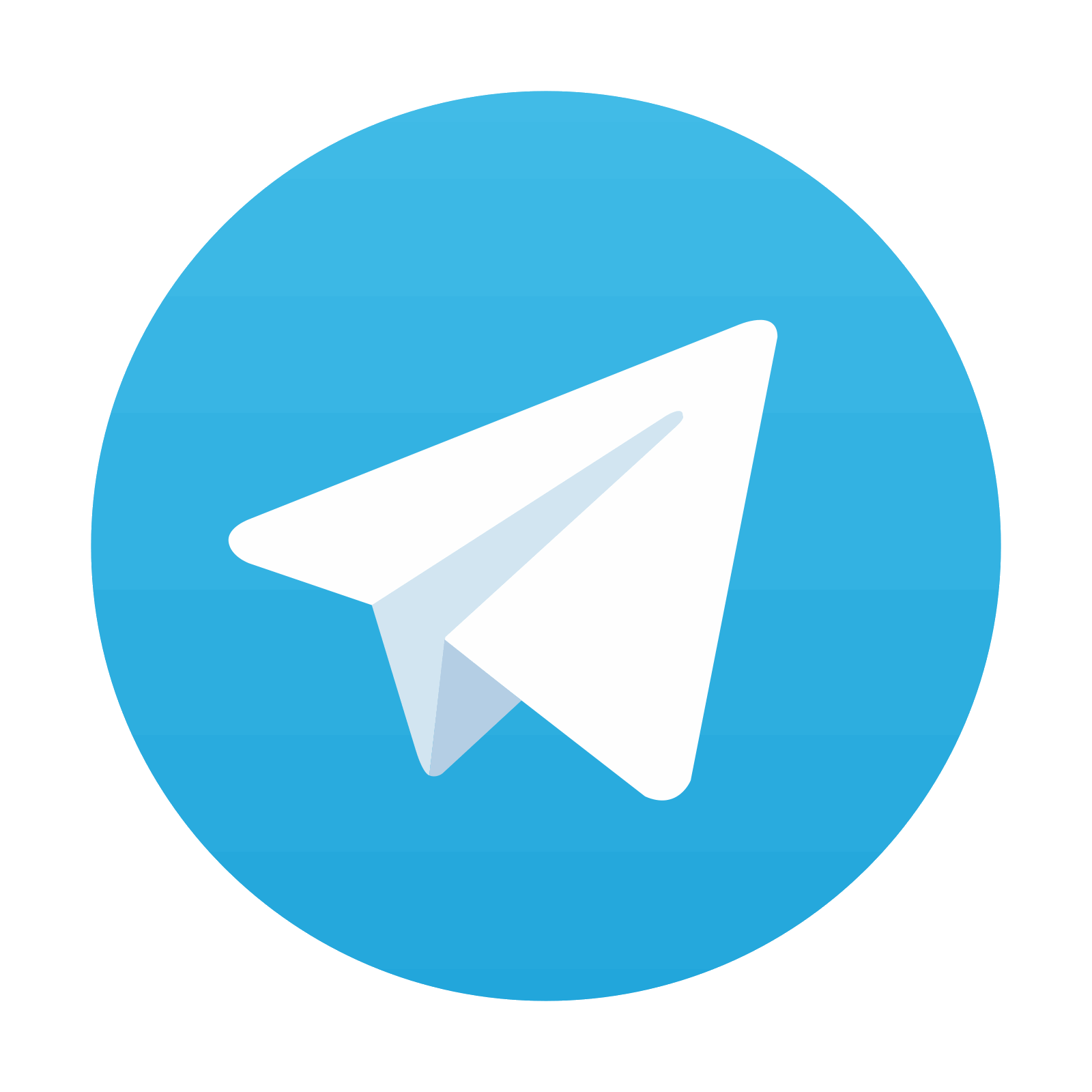
Stay updated, free articles. Join our Telegram channel
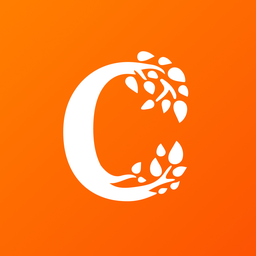
Full access? Get Clinical Tree
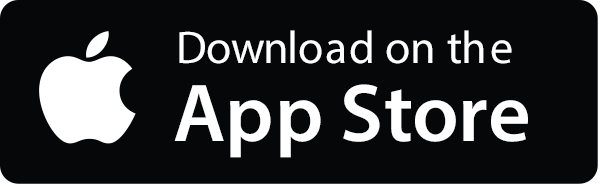
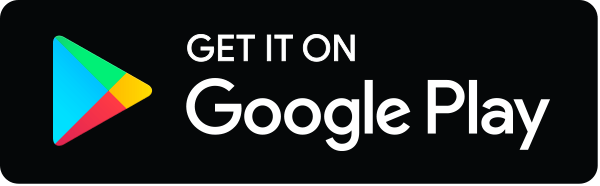
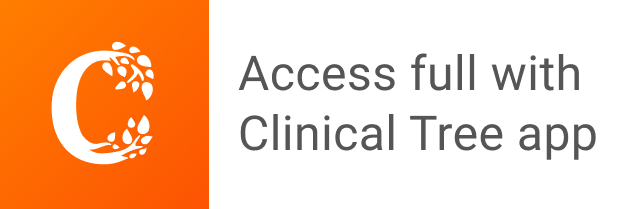