Fig. 76.1
Lungs, airways (including trachea and branching bronchi and bronchioles), and distal alveoli where gas exchange takes place (Modified from http://images.google.com/imgres?imgurl = http://images.webmd.com/images/hw/media65/medical/hw/h5551557.jpg&imgrefurl = http://my.webmd.com/hw/health_guide_atoz/hw141715.asp&h=300&w=460&sz=26&tbnid=jBlE6ZHtW7QJ:&tbnh=81&tbnw=125&hl=en&start=3&prev=/images%3Fq%3Dlungs%2Bairways%26svnum%3D10%26hl%3Den%26lr%3D)
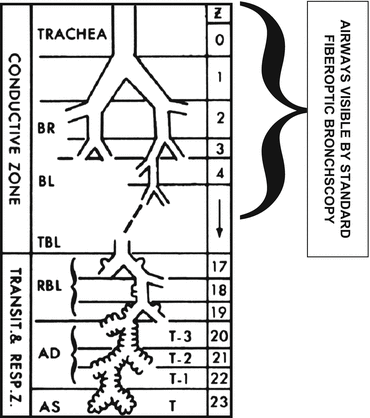
Fig. 76.2
Schematic of airway generations and airway regions accessible for visual examination by flexible fiber-optic bronchoscopy (Modified from Weibel et al. [22])
As with any new technology, the exact clinical role for OCT in pulmonary diagnostics and therapeutics will be defined and evolve over time. Anatomic regions of the pulmonary organ system where OCT may be of clinical or research value include the larynx, vocal cords, proximal airways (including trachea with an average of 1.5 cm2 area and decreasing sized bronchi and bronchioles), smaller gas-exchanging lung structures (including respiratory bronchioles and alveoli of roughly 200–300 μm diameter and 8 μm wall thickness) [20] (Fig. 76.3), and pleural space between the chest wall and lungs (including parenchymal pleura and visceral pleura covering the lung surface) (Fig. 76.4) [15]. In addition, in the pulmonary vascular system including pulmonary arteries, arterioles, more than 300 million alveolar capillary segments (where gas exchange takes place with the blood) [20] (page 13), pulmonary veins, as well as bronchial arteries, capillaries, and veins, OCT may have future applications.
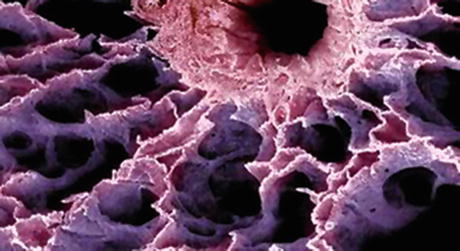
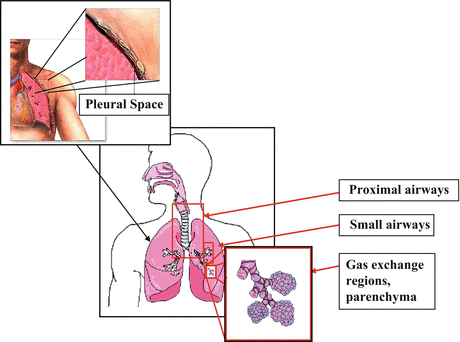
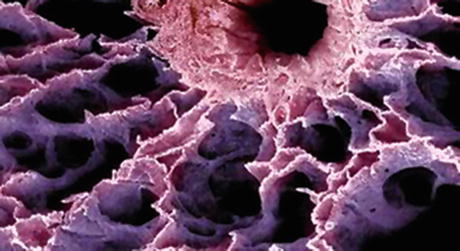
Fig. 76.3
Scanning electron micrograph of bronchiole and distal alveolar structures. Alveoli are approximately 200–300 um in diameter. Fine pulmonary capillary networks perfuse the capillary walls (From http://images.google.com/imgres?imgurl = http://www.visualsunlimited.com/images/watermarked/310/310003.jpg&imgrefurl = http://www.visualsunlimited.com/browse/vu310/vu310003.html&h=251&w=350&sz=28&tbnid=hXOAXFGrrlwJ:&tbnh=83&tbnw=116&hl=en&start=8&prev=/images%3Fq%3Dalveoli%2BSEM%26svnum%3D)
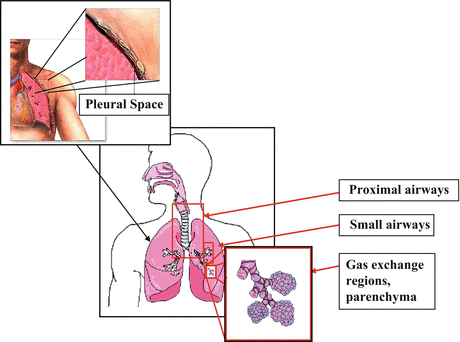
Fig. 76.4
Potential areas for pulmonary OCT clinical and research applications including proximal airways larynx and trachea, bronchi, smaller airways and bronchioles, gas exchanging regions and lung parenchyma, and pleural space (Modified from http://www.smartdraw.com/resources/examples/medical/images/respiratory_full.gif and http://images.google.com/imgres?imgurl = http://www.uscuh.com/CWS/ADAM/Surgery/graphics/Chesttubeinsertion_1.jpg&imgrefurl = http://www.uscuh.com/CWS/ADAM/Surgery/Chesttubeinsertion_1.html&h=320&w=400&sz=13&tbnid=1kw9f2O5YMIJ:&tbnh=96&tbnw=120&hl=en&start=12&prev=/images%3Fq%3Dpleural%2Bspace%26svnum%3D10%26hl%3Den%26lr%3D)
76.3 Standard External Imaging Techniques for Lung and Airway Examination
76.3.1 Chest X-Ray
Chest radiographs are relatively inexpensive, with excellent depth of penetration (the entire thorax can be imaged). Standard chest radiographs are two-dimensional composite shadows of tissue structures, with maximal resolution on the order of millimeters. Digital imaging and subtraction techniques may further improve diagnostic capabilities for chest radiographs and other imaging techniques [28]. Chest radiographs, however, are insensitive for detecting early airway or parenchymal malignancies or structural changes in the airways that can be detected by higher-resolution optical technologies.
76.3.2 Computed Tomography
High-resolution CT scanning is three-dimensional and also has excellent penetration with resolution on the order of hundreds of microns to millimeters [28–30]. Various reconstruction algorithms can be applied including airway surface renderings for “virtual” “bronchoscopy” [29, 31–33]. However, virtual bronchoscopy is limited in ability to evaluate the mucosal surface of the respiratory tract reliably. Although the overall surface form can be detected, mucosal color, irregularity, or friability cannot be assessed [33]. Studies show that CT imaging detects changes in airway wall thickness in obstructive airway disorders, and these correlate with disease severity and bronchial biopsy measurements of airway wall thickness [34]. However, beyond the fifth airway generation, CT’s resolution is inadequate, and in addition, CT cannot detect or measure morphological changes in the airway structured microlayers. These limitations are potentially overcome by optical technologies.
76.3.3 Positron Emission Tomography
Positron emission scanning is relatively low resolution but provides functional information that may be useful in differentiating benign from malignant pathology, particularly in conjunction with CT scanning [35, 36]. PET scanning may be particularly useful for detection of unsuspected metastatic malignant disease in lung cancer patients [35]. As a functional imaging modality, integrated chest PET CT scanning is currently used in diagnostic algorithms for indeterminate pulmonary nodules and staging of cancers.
76.3.4 Magnetic Resonance Imaging
MRI imaging is rapidly advancing for thoracic imaging with improvements in acquisition times but is used primarily for mediastinal and proximal vascular structure evaluation in the thorax at this time [28, 30]. MRI is used occasionally in tracheobronchomalacia and for detecting involvement of the chest wall structures by tumor [30, 37]. The depth of penetration is throughout the thorax, and imaging resolution is on the order of millimeters [29].
76.4 Direct Examination Techniques for Airways, Lung Parenchyma, and Pleura
76.4.1 Flexible Bronchoscopy
Flexible bronchoscopy is performed either trans-orally or trans-nasally using flexible endoscopes that are usually at least 100 cm in length and have outer diameters ranging from 3 to 6.5 mm. The bronchoscopes are now commonly videoendoscopes. The bronchoscopes most commonly have a light source, imaging modality (CCD chip or fiber bundle), and a hollow “working channel” usually ranging from 1.2 to 2.8 mm. Specimens can be obtained including suctioning, saline lavage, brushing, and needle and forceps biopsies (generally of maximal diameter on the order of 1–2.5 mm). The working channels allow the passage of therapeutic instruments (laser, electrocautery, cryotherapy probes) or novel diagnostic tools such as OCT, radial EBUS, or CFM probes. Generally, 3–4 orders of bronchial branching can be directly visualized with standard flexible bronchoscopy, but ultrathin flexible bronchoscopy using flexible scopes of 2–3 mm and working channels of 1 mm allow access to more distal airways and pulmonary abnormalities peripherally situated within the lung parenchyma. The major advantage of flexible bronchoscopy is its versatility. Flexible bronchoscopy can be performed in awake patients with or without moderate sedation. Its use is easily learned, and it enables the operator to reach the distal aspects of branching airways with minimal trauma or patient discomfort. Diagnostic procedures, including those with biopsies, can usually be performed in less than 10 min. Procedures can be performed using topical anesthesia, and bronchoscopy can be done at the patient’s bedside or in an outpatient setting. White light bronchoscopy, however, can only image mucosal surfaces. For cancer, for instance, even for visible endobronchial lesions, combined bronchoscopic modalities (brushings, washings, biopsy) result in a diagnostic rate that is less than 100 %, mainly because of sampling errors (biopsies are performed from areas of tumor necrosis, inflammation, or fibrosis). High-magnification bronchoscopy (HMB) provides information of the bronchial mucosa with a maximum magnification of 110 times, thus revealing subepithelial microvessels in the large airways, potentially useful to abnormal vascular networks in the large airway in asthma and lung cancer [38, 39]. HMB, however, is still a surface imaging modality with a resolution of approximately 20 μm. Many pathologic subepithelial vessels have a diameter less than that; in addition, HMB cannot distinguish neovascularization from vasodilation [40].
76.4.2 Rigid Bronchoscopy
Rigid bronchoscopy is usually performed in conjunction with interventional therapeutic procedures. These include laser resection, tumor debulking, silicone and metal stent insertion, or dilatation of benign tracheal or bronchial strictures. Rigid bronchoscopes generally range in size from 5 to 12 mm diameter and 12 to 45 cm in length. Working channels can be as large as 7 mm in addition to the visualization optics. Rigid bronchoscopes can visualize up to four orders of bronchial branching and are primarily focused on proximal airways. Visualization is direct, and resolution is governed by the quality of Hopkins lens rigid telescopes. Airway surface anatomy is visualized with rigid bronchoscopy usually using straight lens telescopes with a digital or other high-grade video camera easily attached to the telescope head. Lateral viewing telescopes of 30, 45, and even 90° can be used, but are infrequently employed today because of the ready availability of the flexible bronchoscope. It is noteworthy that probes or catheters placed through the rigid tube need to be sufficiently firm so that they can be manipulated inside the open tube (Fig. 76.5). This is the case, for example, for existing silicone suction catheters, quartz laser delivery fibers, OCT probes, or radial EBUS (Fig. 76.6). In addition to limitation of white light bronchoscopy, rigid bronchoscopy may restrict the wide application of novel optical technologies in pulmonary medicine because of its limited applications and availability among pulmonologists. Furthermore, the systems can be cumbersome to use in conjunction with dedicated OCT probes, since through a hollow tube, the operator has to accommodate the telescope and the OCT probe and sometimes forceps and laser fibers (Fig. 76.7). This limitation, however, can be potentially overcome by development of integrated WLB-OCT laser systems.
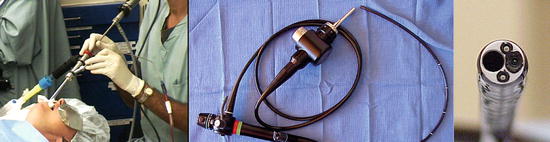
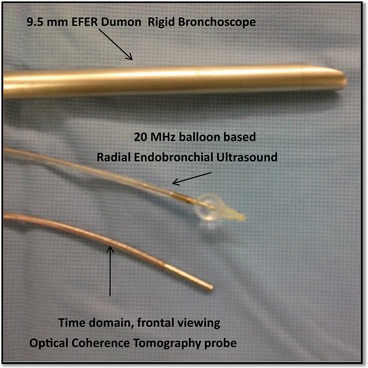
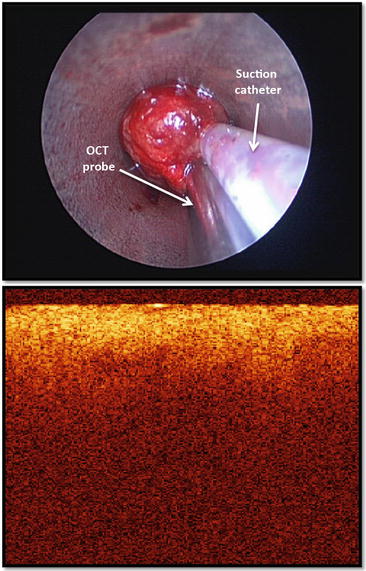
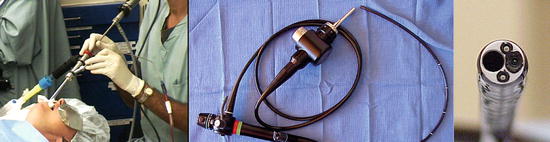
Fig. 76.5
(Left) 12 mm diameter rigid bronchoscope (Bryan Corp, Woburn MA, USA). Note 5 mm rigid telescope, attached digital camera, 3 mm suction catheter placed through sideport and black flexible Racine connector allowing mechanical ventilation and oxygenation. (Center) Flexible videobronchoscope (Olympus, Tokyo JP) (From The Essential Bronchoscopist© bronchoscopy.com.uci.edu). (Right): Flexible fiber-optic bronchoscope seen on end. Note 2.8 hollow working channel)
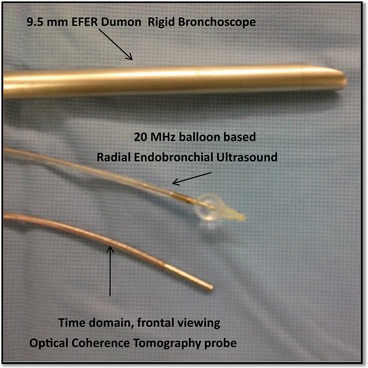
Fig. 76.6
The rigid bronchoscope accommodates a variety of instruments including the telescopes, forceps, suction catheters, laser fibers, as well as optical and ultrasound imaging probes. This is a close view of the 9.5 mm EFER-Dumon rigid bronchoscope, the radial ultrasound probe with its water-filled balloon and the optical coherence tomography probe
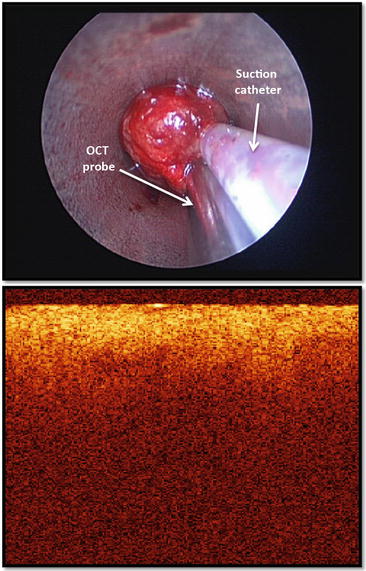
Fig. 76.7
The suction catheter and a time domain forward viewing OCT probe are placed over an endobronchial tumor just before its removal from the airway (top panel). The OCT tomogram revealed a pattern of homogeneous light backscattering layer and absence of normal layered microstructures which characterize the normal airway wall (bottom panel)
76.4.3 Autofluorescence Bronchoscopy (AFB)
The main rationale for the development of AFB is the fact that WLB is not sensitive enough for detecting abnormalities that are only a few cells thick or below the tissue surface. AFB is based on the hypothesis that differences in epithelial thickness, blood flow, and tissue fluorophore concentration cause abnormal tissues to have diminished red and substantially diminished green fluorescence as compared to normal tissues when exposed to 440–480 nm wavelength blue light excitation (Fig. 76.8); thus, autofluorescence (AF) cannot be seen during conventional WLB as the intensity of fluorescence is very low and is overwhelmed by the reflected and backscattered light. Successful localization of a dysplastic lesion is possible using AFB, whereas WLB is normal. The intensity of green fluorescence is measured, and an abnormally low “green” fluorescent area is identified as a cold spot on a normal green background. The published evidence shows that AFB has a better sensitivity than WLB for detecting intraepithelial lesions [41]. The specificity, however, is low (~60 % vs ~90 % for WLB) due to false-positive fluorescence with inflammation, mucosal gland hyperplasia, and interobserver error. To increase specificity, one may need to obtain information about tissue structure (via OCT or CFM) [24].
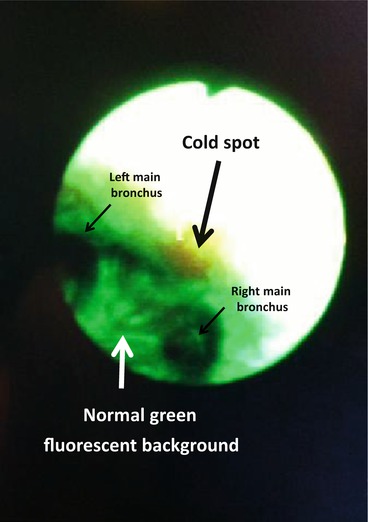
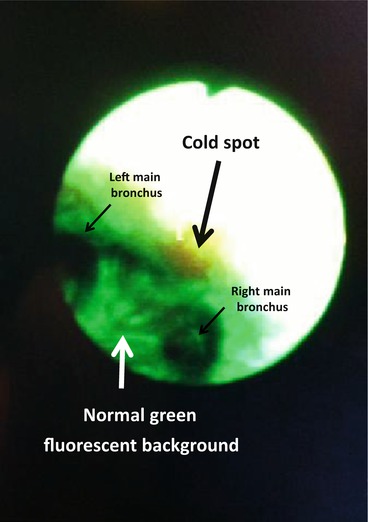
Fig. 76.8
Abnormal tissues have diminished green fluorescence as compared to normal tissues when exposed to 440–480 nm wavelength blue light excitation. The intensity of green fluorescence is measured, and an abnormally low “green” fluorescent area is identified as a “cold spot” on a normal green background (arrow)
76.4.4 Narrow Band Imaging (NBI)
The rationale for development of NBI relies on the fact that malignant and dysplastic lesions are characterized by abnormal angiogenesis which can be detected visually as vessel entanglement and abnormal proliferation within the bronchial mucosa [42]. NBI uses blue light centered at 415 nm and green light centered at 540 nm corresponding to the maximal hemoglobin absorption peaks. The blue light highlights the superficial capillaries, whereas the green light can penetrate deeper and highlight the larger blood vessels in the submucosa (Fig. 76.9). Preliminary studies suggest that NBI can improve detection of dysplasia and CIS compared with WLB [43, 44]. The routine clinical utility of NBI, however, remains unknown as the natural history of the above premalignant lesions is not completely well understood, the indications for NBI have not been well established, and inter- and intra-observer reliability recognizing normal and abnormal NBI images needs to be further defined.
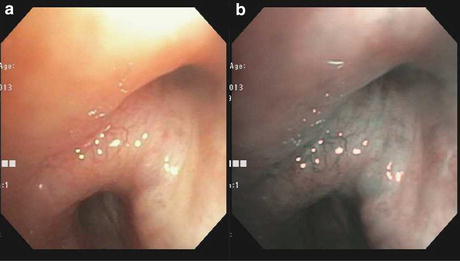
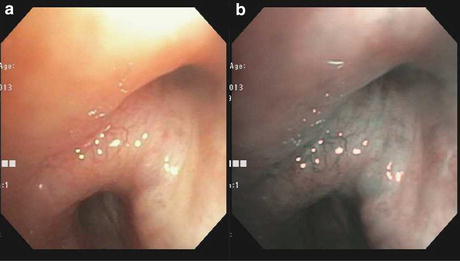
Fig. 76.9
(a) White light bronchoscopy at the level of primary right carina (RC1) shows submucosal tumor infiltration. (b) Narrow band imaging at the same anatomic site: the blue light highlights the superficial capillaries, whereas the green light can penetrate deeper and highlight the larger blood vessels in the submucosa
76.4.5 Confocal Endomicroscopy (CEM)
Confocal endomicroscopy (CEM) provides an image of a thin section within a biological sample, where the microscope’s objective is replaced by a flexible fiber-optic miniprobe [45]. CEM offers spatial resolution down to the submicron range and depth of penetration of approximately 0.5 mm, which should provide sufficient optical information to distinguish benign from early malignant lesions since cellular changes in CIS and dysplasia occur in less than 1 mm in depth, if optical contrast is sufficient. The basement membrane and upper submucosa can be imaged with good quality. Disorganization of the fiber network was seen indicating basement membrane disruption that was observed in the majority of metaplastic and dysplastic samples and all CIS and invasive cancers [45]. CEM has been also investigated in vivo for studying airway remodeling in asthma [46]. While there was no difference in CEM patterns between asthma patients and healthy controls, there was an association between post-bronchodilator FEV1 % predicted and both histologic and CEM elastic fiber patterns. These findings suggest there may be a structure-function relationship between extracellular matrix in the airway wall and lung function. Because cells do not emit strong autofluorescence with the current commercially available technology, the epithelial cells are not visible. In addition, the fragile epithelium can be scrapped off during the imaging procedure. Studies are needed to evaluate if CEM can distinguish normal from specific abnormalities (dysplasia, inflammation, fibrosis, malignancy), not just normal from abnormal tissues [47]. Furthermore, since the depth of penetration is limited, this technique will likely be appropriate in a multimodality bronchoscopic imaging platform, combining it with technologies able to visualize the cartilage and its potential invasion (i.e., EBUS) and reveal alterations in the airway wall microstructure (i.e., OCT).
76.4.6 Pleuroscopy
Pleuroscopy is a procedure that allows physicians to access to the pleural space. It is also known in the literature as thoracoscopy, medical thoracoscopy, or video-assisted thoracic surgery (VATS). In general, however, VATS refers to thoracoscopy performed in the operating room using single-lung ventilation. During medical thoracoscopy, the operator (surgeon or interventional pulmonologist) can perform parietal pleural biopsies, drainage of pleural effusions, and pleurodesis either in an endoscopy suite or operating room. In addition to these procedures, during VATS, thoracic surgeons can also perform lung biopsy, resection of pulmonary nodules or blebs, lobectomy, pneumonectomy, or esophagectomy [48]. Either rigid or flexible instruments can be used for these interventions, but surgeons use mostly rigid instrumentation. Disposable plastic or reusable stainless steel trocars come in different sizes (5–13 mm) and allow the passage of telescopes with different angles of vision (0° for direct viewing and 30–50°) for oblique panoramic viewing of the pleural cavity. In addition, other instruments such as forceps or optical probes can be passed through the trocar inside the thoracic cavity and potentially assist with selection of a biopsy site (Fig. 76.10). The flex-rigid pleuroscope (model LTF 160 or 240; Olympus, Tokyo, Japan) is designed like a bronchoscope with a handle and a shaft of 7 mm outer diameter. The 2.8 mm working channel accommodates forceps, electrosurgical and laser probes, and other accessories (Fig. 76.10). The yield of thoracoscopic parietal pleural biopsy for malignant pleural effusions is 90–100 % [49]. It is possible that the addition of optical technologies will maximize this yield, especially for early-stage mesothelioma, which could resemble pleural inflammation. Overall, however, the limited published clinical evidence available to date does not support the use of autofluorescence or NBI in addition to white light thoracoscopy for the diagnosis of malignant pleural effusion [50, 51].
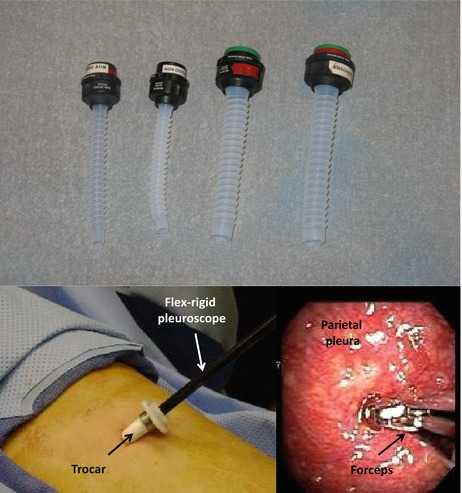
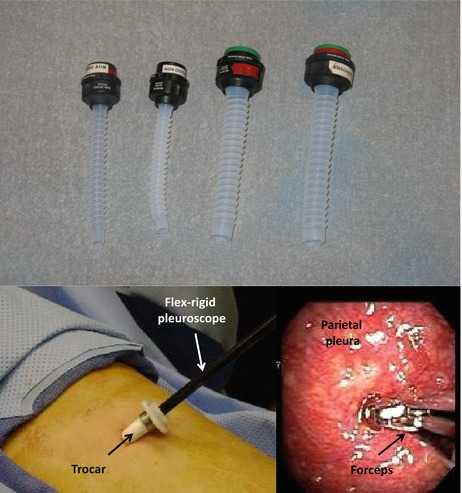
Fig. 76.10
Trocars allow insertion of various instruments (top panel). The 2.8 mm working channel of the flex-rigid pleuroscope accommodates forceps, electrosurgical and laser probes, and other accessories (bottom panel)
76.5 Anatomic Regions of the Pulmonary System Amenable to Potential Clinical OCT Applications
76.5.1 Pulmonary Airway Tree
OCT has been demonstrated in a number of studies to acquire images with sufficient contrast and resolution to reliably identify many of the normal architectural features of the airway, parenchyma, and pleura. Deviations from this normal structure such as the loss of the layering of the airway wall, or the visibility of alveoli in the parenchyma, may be indicative of the presence of a pathologic abnormality. The initial reports of OCT in pulmonary medicine involved studies of normal and abnormal tracheal, bronchial, and bronchiolar anatomy in excised tissue specimens [10, 12–14, 52]. The ability of OCT to differentiate epithelial, subepithelial, glandular, cartilaginous, and muscular structures within the trachea of humans and animal models was demonstrated. However, the development and validation of accurate image interpretation criteria to reliably assess OCT images of pulmonary tissues obtained in vivo is a necessary step if OCT is to be adopted in the clinical setting. A number of groups are assessing the close similarity between OCT imaging and standard histologically stained excised specimens (Figs. 76.11, 76.12 and 76.13) [10, 12, 15, 16, 24, 53] and are now beginning to use this information for develop interpretation guidelines (Table 76.1) [53].
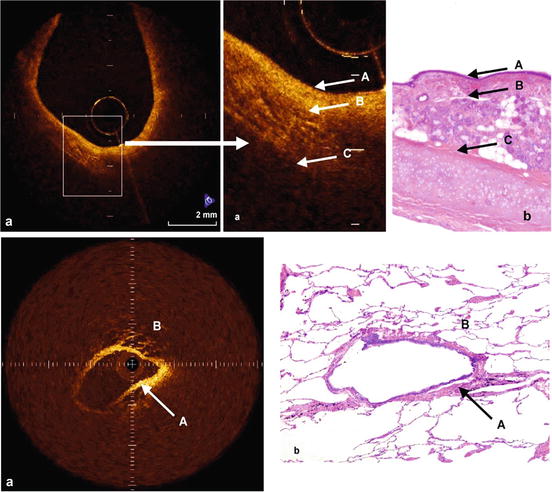
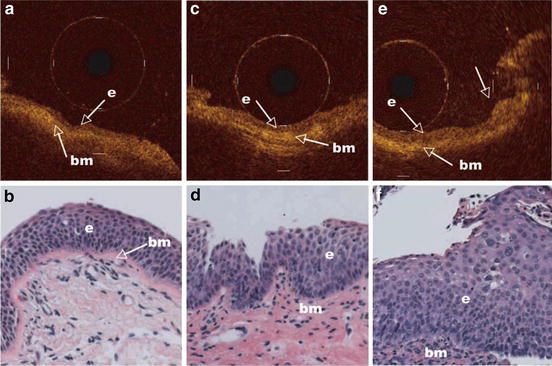
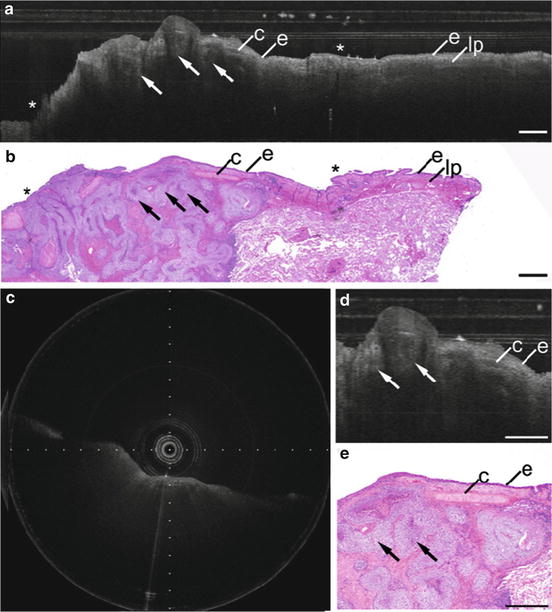
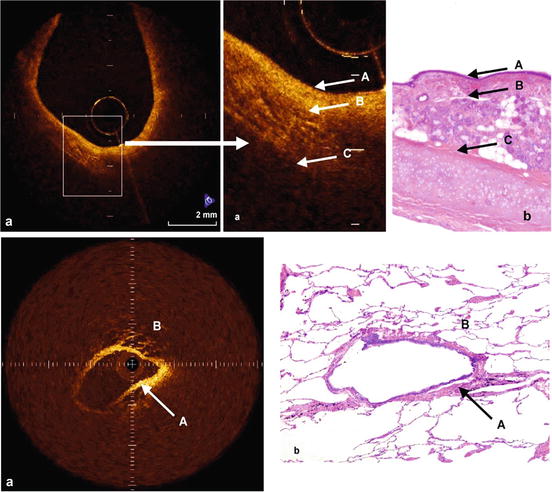
Fig. 76.11
Rotational probe images from human lung specimens compared to corresponding histology from Tsuboi [10]. Images obtained from a SLD laser system (1,300 nm center λ, 70 nm bandwidth). Top image shows the OCT image (a), and histological finding (b), of normal bronchus. The bronchial mucosal and submucosal layer (A) appear homogenous in OCT image. The submucosal layer is relatively reflective due to the presence of extracellular matrix. A gap can be seen between the submucosa (A) and smooth muscle layer (B), and cartilage layer shows much scattering (C). Epithelium, mucosa, and cartilage are clearly differentiated, as well as a number of glandular tissues and microvessels by both OCT and histology. (a) OCT images, (b) histological finding, (A) mucosal and submucosal layer, (B) smooth muscle layer, (C) cartilage. Bottom figures show the OCT image (a), and histological findings (b), of normal peripheral bronchus and alveoli. The uniform bronchial wall (A), and the structure of alveoli (B), containing air can be observed. (a) OCT images, (b) histological finding, (A) peripheral bronchus, (B) alveoli (From [10])
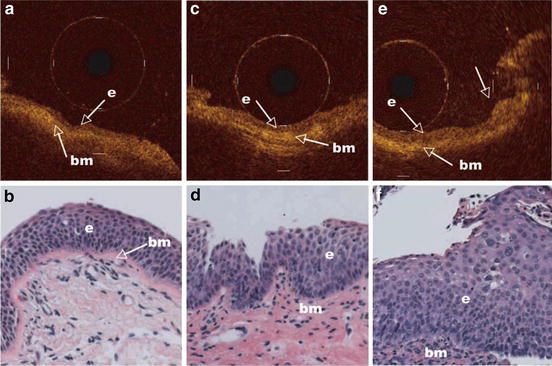
Fig. 76.12
Representative OCT images of an area with mild dysplasia (a), moderate dysplasia (c), and severe dysplasia (e) and corresponding H&E-stained histologic sections (b, d, and f; original magnification, ×20). The nuclei in the epithelium become recognizable as darker dots with moderate dysplasia or worse. The arrow in the OCT image of the area with severe dysplasia points to the corresponding elevated area in the H&E section (Reprinted with permission from [24])
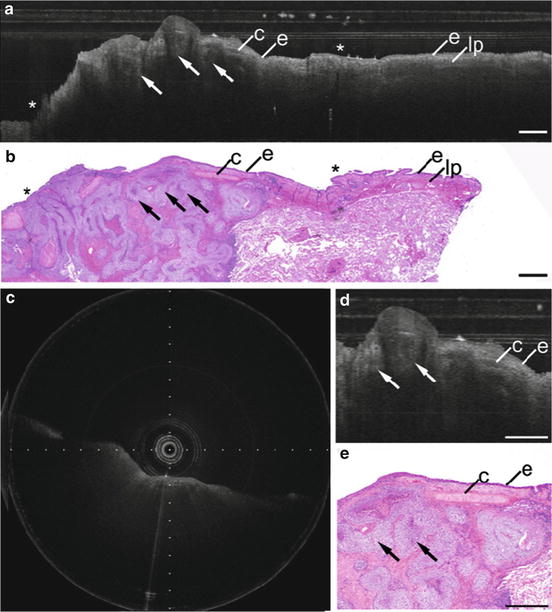
Fig. 76.13
Bronchoscopic catheter-based OCT of squamous cell carcinoma, baseloid type. (a) OCT longitudinal section and (b) corresponding histology. (c) OCT cross section. (d, e) Higher magnification OCT longitudinal section and corresponding histology. e epithelium, lp lamina propria, c cartilage, Arrows nests of hyperintense squamous cell carcinoma with signal-poor central necrosis, Asterisks ink marks for registration. Scale bars 1.0 mm. (Reprinted with permission from [53])
Table 76.1
OCT image interpretation features for benign and malignant pulmonary pathology (reprinted with permission [53])
Pathology | Imaging features |
---|---|
Normal | |
Airway | Layered architecture, including |
Respiratory epithelium and underlying basement membrane | |
Lamina propria | |
Cartilaginous rings | |
Parenchyma | Thin, lattice-like alveolar architecture with signal void spaces |
Neoplastic | |
Adenocarcinoma | Loss of layered architecture |
Mass with signal heterogeneity with rapid attenuation | |
Atypical gland formation | |
Mucinous adenocarcinoma | Signal intense alveolar wall thickening |
Preserved lattice-like architecture | |
Signal poor intra-alveolar mucin | |
Homogeneous signal intense tumor-associated scar may be seen | |
Squamous cell carcinoma, basaloid type | Loss of layered architecture |
Mass with signal heterogeneity with rapid attenuation | |
Irregular tumor nests with: | |
Signal-intense periphery corresponding to malignant cells | |
Signal-poor center corresponding to central necrosis | |
Salivary gland adenocarcinoma, adenoid cystic carcinoma | Luminal mass protrusion |
Uniform distribution of signal-intense trabeculae | |
Small pseudocystic spaces filled with mucin can be seen | |
Intact overlying respiratory epithelium may be present | |
Benign | |
Cartilaginous hamartoma | Lobulated mass with well-delineated borders and |
Evenly dispersed, fine-scale regions of high signal intensity | |
Background of uniform, moderate signal intensity | |
Fibrosis | |
Usual interstitial fibrosis | Diffuse, signal dense subpleural fibrosis |
Irregularly shaped, signal poor subpleural cysts | |
Focal mild subpleural fibrosis, idiopathic | Focal, signal dense pleural fibrosis |
Interspersed lattice-like alveoli with mildly thickened alveolar walls |
These OCT features can be applied to a multitude of benign and malignant processes in the large and small airways. In addition to airway cancer, OCT can be used to study airway wall thickness and remodeling in asthma, COPD, and bronchiectasis [25, 26]. Long-range OCT applications allow the study of airway compliance and potentially facilitate the identification of flow limiting segments in asthma, COPD, tracheomalacia, and obstructive sleep apnea [54–58]. This is clinically relevant for several reasons: (1) it facilitates airway selection for novel bronchoscopic asthma treatments (bronchial thermoplasty); (2) leads to better understanding of central airway mechanics (elastic properties, dynamic compression) in asthma and COPD; (3) allows measurement of the central airway lumen at normal and stenotic sites, potentially guiding bronchoscopic interventions; and (4) facilitates quantitative evaluation of the upper airway dynamics and localization of the sites of obstruction during polysomnography or continuous positive airway pressure thus potentially guiding surgical interventions for OSA.
76.5.2 Lung Parenchyma
OCT can potentially be used to study focal or diffuse lung parenchymal processes. Current research is limited to ex vivo studies aiming to understand normal and pathologic alveolar structure and dynamics [1, 59]. Current limitations in clinical applications include technical challenges due to the invasive access to the alveolar space (may require pleuroscopy) and OCT imaging itself of the alveoli due to changes in the refractive index of air, tissue, and mucus (may require liquid filling index matching). Potential clinical applications would likely require a bronchoscopic approach in which the OCT probe is guided to a target previously identified on computed tomography. This is now possible using electromagnetic navigation bronchoscopy, which provides a three-dimensional virtual road map that enables a physician to maneuver through multiple branches of the bronchial tree. Once the desired location is achieved, the insertion of a needle probe could allow OCT imaging and biopsy of the desired region (i.e., focal lung mass or alveolar space) [60–62]. In addition, one could gain more information about the basic alveolar dynamics during normal breathing and artificial ventilation [63]. This information could potentially be used to prevent alveolar damage and guide protective lung ventilation strategies in patients with acute respiratory distress syndrome (ARDS), though this is speculative at this time [64].
76.5.3 Pleura
The pleural space is a virtual space between the lungs and their surrounding thoracic structures including the chest wall, mediastinum, and diaphragmatic surface (Fig. 76.4). The pleural space is a site of important thoracic pathology including primary malignancies, metastatic malignancy, fluid collections (benign and malignant), infection, and traumatic and inflammatory conditions. Accurate diagnosis of pleural disease may require open thoracotomy or thoracoscopic examination. Detecting malignancy in the pleural space is essential for proper staging and management of lung cancers. Diagnostic yield from thoracentesis (pleural fluid drainage) is often low. Yield from thoracoscopy is substantially higher. It is uncertain at this time whether OCT will have a role in pleural diagnostics, though it is reasonable to speculate that the ability to obtain high-resolution functional and structural characteristics of pleural spaces and surfaces prior to biopsy would be of potential benefit. This may particularly apply to mesothelioma for which the pleural findings on white light imaging may be nonspecific and deeper pleural biopsies are usually necessary for diagnosis. It is likely that if OCT will have a role in pleural investigations, it will be in a multimodal approach in conjunction with white light thoracoscopy and potentially NBI or AF. OCT may be particularly useful in guiding the site of thoracoscopic biopsy, if studies will confirm that tumor can be distinguished from adjacent nonmalignant findings. Current research in OCT imaging of the pleura is limited to animal studies which demonstrate that fine structures such as the visceral pleura and alveoli were able to be visualized with OCT at high resolution, but it remains to be determined whether OCT is capable of differentiating between benign and malignant pathologies [15, 65].
76.5.4 Pulmonary Vascular Tree
Pulmonary vessels can be accessed for OCT imaging at the time of right heart catheterization. The pulmonary vascular wall changes due to hypertrophy of the intima and media (such as in pulmonary arterial hypertension) or detection of flaps inside the lumen (due to thrombus) can be detected by OCT. This could have diagnostic and consequent therapeutic implications since OCT can potentially distinguish chronic thromboembolic hypertension from other etiologies [66]. Technically, however, application techniques for OCT imaging of the pulmonary vasculature are not obvious since it may require proximal balloon occlusion and injection of saline or lactated Ringer solution to facilitate penetration of light. These limitations have already been addressed in intracoronary OCT imaging where increases in image acquisition times have negated the need for proximal balloon occlusion and require only a very short saline, lactated Ringers solution, or radiocontrast flush (<10 mL total). Therefore, it is feasible that with further investigation and refinement of imaging techniques, these issues may additionally be overcome in the pulmonary vasculature.
76.6 Pulmonary OCT Imaging Systems and Approaches
76.6.1 Endobronchial
The use of OCT to study the lung in the clinical setting has been predominantly restricted to assessment of airways within the tracheobronchial tree that are accessible with a standard bronchoscope. Endobronchial OCT imaging is typically performed by inserting the OCT catheter through the accessory port of a standard bronchoscope (Fig. 76.14) [10, 24, 53, 67]. Alternative placement of catheters into the more distal airways may be achieved using CT, or fluoroscopic guidance, or by using a guide sheath in conjunction with electromagnetic guidance techniques.
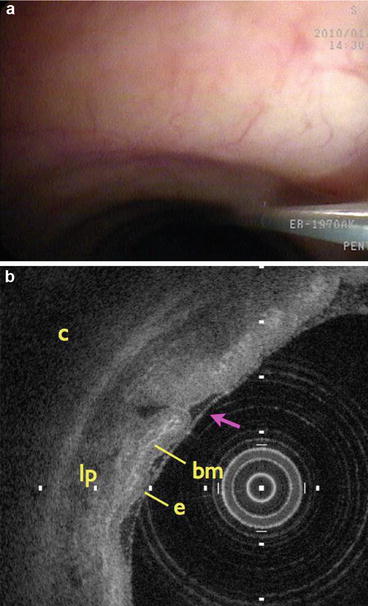
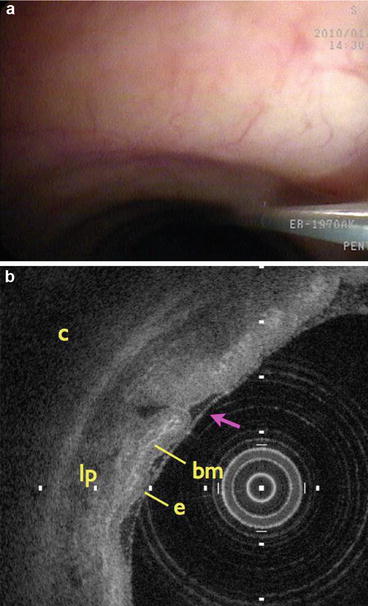
Fig. 76.14
Clinical endobronchial OCT imaging. (a) Spiral cross-sectional OCT catheter extending through the accessory port of a standard bronchoscope into the airway lumen. (b) Cross-sectional OCT image highlighting the normal layered architecture of the airway wall. e epithelium, bm basement membrane, lp lamina propria, c cartilage
Endobronchial OCT imaging may be performed using a variety of catheter designs. Prior studies have included both circumferential [10, 24, 41, 54] and linear scanning [15, 16] mechanisms, which may be actuated either proximally outside the patient or at the distal tip of the catheter using miniature motors [68] or galvanometric scanners. The designs of both linear and circumferential catheters that are actuated proximally typically consist of an optical inner core encased inside an optically transparent sheath. The primary purpose of the sheath, which is typically single use, is to protect the patient from contact with the reusable inner optical core and to prevent contamination of the optical core with bodily fluids. A common design of the inner optical core consists of a fiber to transmit the OCT signal, coupled to a focusing element which may consist of a gradient index lens [15], ball lens, or other focusing element and a reflecting surface such as a prism to redirect the light to the tissue surface at an angle of approximately 90° to the catheter. The entire optical assembly is encased inside a metal drive shaft to provide added protection to the optical components against breakage and to convey the necessary translational actuation from the proximal end during tissue scanning. The primary difference between linear and circumferential scanning catheters is that the proximal end of circumferential scanning catheters is attached to an optical rotary junction. Together both actuation methods can be used to achieve spiral cross-sectional imaging to capture volumetric images of entire bronchial segments. While many scanning mechanisms may be used to collect OCT images of the airways, circumferential and spiral cross-sectional scanning catheters have been used predominantly for clinical studies in order to acquire complete cross-sectional imaging of the entire airway lumen.
76.6.2 Transbronchial and Transthoracic
In order for OCT to play a future role the evaluation of lung pathology and the guidance of biopsy acquisition, access to regions of the lung that extend beyond the central airways is necessary. This may be through transbronchial approaches where a needle or catheter is passed through the airway wall in order to reach the surrounding parenchyma or through transthoracic approaches where a needle or catheter is passed through the chest wall into the lung. Although no transbronchial or transthoracic OCT catheters are currently available for clinical use, a number of groups are actively working on the development of these much needed imaging tools.
A number of small diameter rigid OCT needle probes have been developed by research laboratories that may be adapted for transthoracic use [69–73]. In these designs the imaging optics are traditionally housed within the stainless steel hypodermic needles to ensure that the needle probe maintains a small diameter profile to minimize possible tissue damage. To conduct imaging the entire needle is rotated or translated. In many potential clinical applications of transthoracic OCT imaging, it may be useful to obtain biopsy specimens for histopathological analysis prior to imaging. Therefore, one limitation to these OCT needle probes is that it is not possible to obtain tissue specimens for diagnosis through the same imaging needle. Recently, Kuo et al. demonstrated an OCT-guided core-needle biopsy system incorporating a vacuum-assisted rigid breast needle biopsy console, which was modified to accommodate the OCT imaging probe [61]. This work highlights the potential of OCT image guidance for biopsy site selection with rigid needle designs.
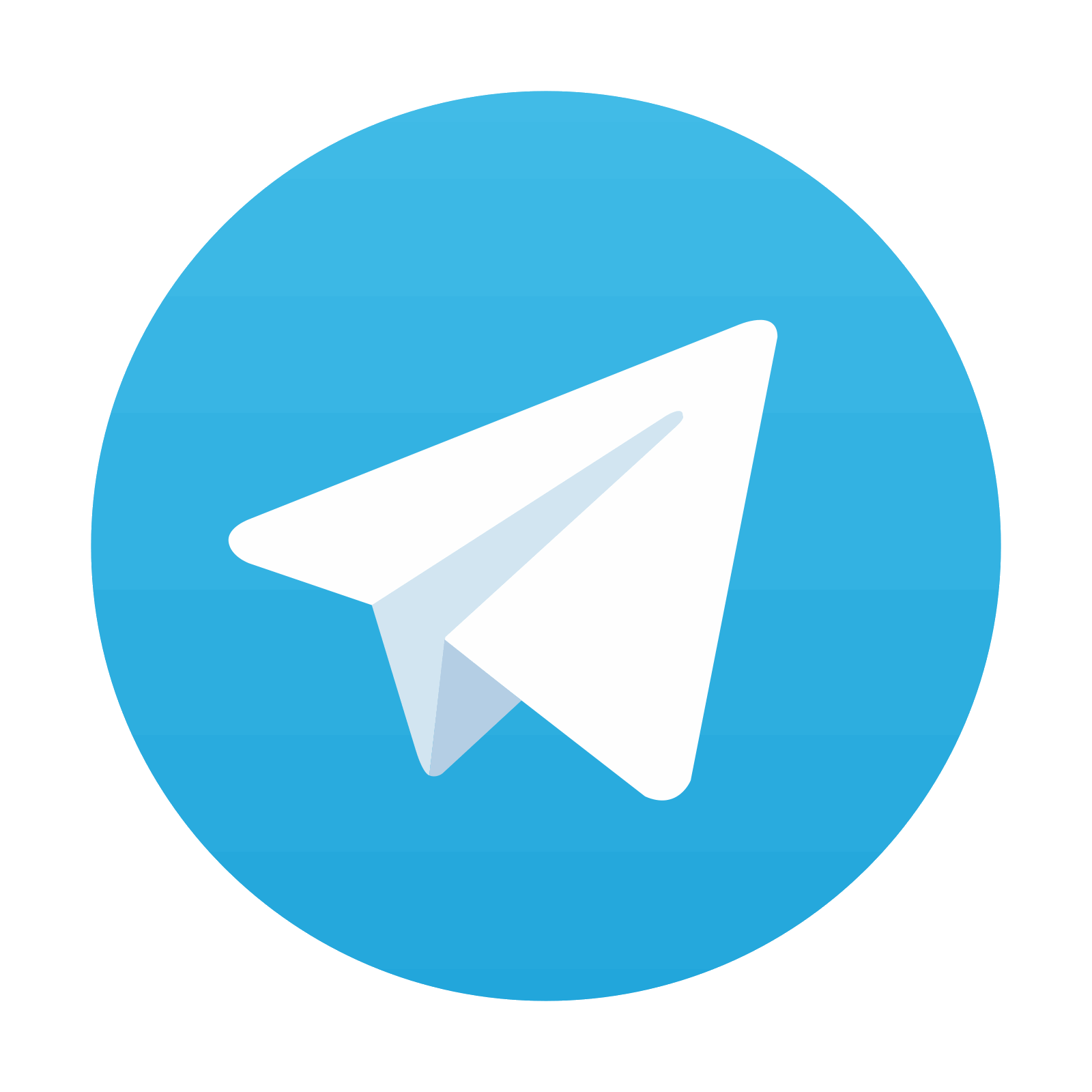
Stay updated, free articles. Join our Telegram channel
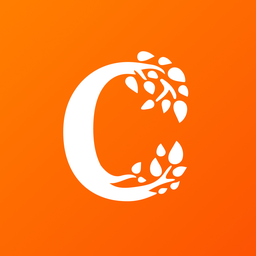
Full access? Get Clinical Tree
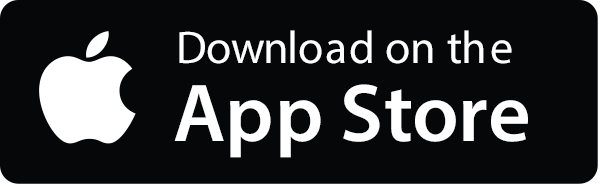
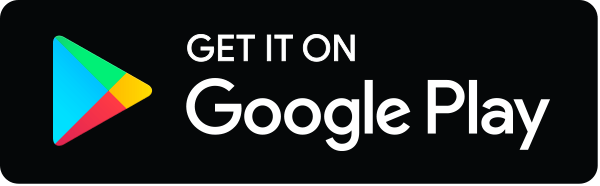