Fig. 45.1
Longitudinal OCT-based vascular imaging of a breast cancer (MCaIV) tumor growing in a dorsal skinfold chamber (angiography). Color is used to encode vessel depth in these projections of the three-dimensional angiographic dataset. Relative imaging times: (a) d0; (b) d2; (c) d6; (d) d10
To detect OCT signal dynamics, at least two measurements of nominally the same location are compared. Original approaches used adjacent depth scans (A-lines) for these measurements and slowed the transverse beam scanning to ensure sufficient beam overlap between the measurements [22–24]. While this approach is straightforward to implement, the time separation between measurements was relatively small and dropped as OCT A-line rates increased. This resulted in relatively poor flow sensitivity, yielding patchwork angiographic datasets characterized by frequent missing vessels and vessel segments. The sensitivity of OCT-based angiography to smaller and slower flowing vessels increased dramatically when angiographic imaging adopted beam scanning sequences that decoupled the time difference between repeated measurements from the system A-line rate. This could be done as simply as comparing between frames (thereby linking measurement timing to the frame rate) [26, 27, 30, 36] or by designing more complex scan patterns that separate both the frame rate and the A-line rate from the measurement timing [6, 29, 37].
Animal motion is a persistent challenge for preclinical angiographic imaging. Bulk physiological motion from animal respiration or cardiac cycles can induce submicron displacements which are easily detectable by OCT and can obscure vascular signals. Two strategies are combined to limit bulk motion degradation: mechanical stabilization and algorithm design. Mechanical approaches simply limit the magnitude of physiological motion. These include the stable fixture of window frames (dorsal or mammary fat-pad window models [21]) to the microscope, or a secure holding of the animal head when imaging in the brain. Imaging in the skin and with subcutaneous (non-window) models typically requires some level of mechanical stabilization; otherwise, respiratory motion often dominates vascular signals. Adhesive fixtures [30] or inverted microscope designs can be used in these studies. However, when immobilizing the imaging area, it is important to control the pressure in order to maintain blood flow and avoid possible occlusions. The second strategy to combat bulk motion is algorithmic in nature. Many of the most successful angiographic algorithms are designed to be inherently robust to sub-pixel axial displacements induced by environmental fluctuations and animal motion. While automated measurement and removal of this motion is possible in some applications, robust deployment in large biological studies benefits from a more fail-proof method to limit bulk motion sensitivity. Eliminating OCT signal phase [30–32], or specifically tailoring how phase data is included in angiographic processing [6], has yielded algorithms that are intrinsically insensitive to sub-resolution axial bulk motion.
45.2 Tumor Margins
Tumor margins are extremely important not only in cancer investigation but also in the clinic. The boundaries between healthy and tumor tissue are often blurred and, in most cases, difficult to distinguish in vivo with current imaging diagnostics without the need for a biopsy. In recent years, the study and characterization of the tumor microenvironment and the delicate, complex dialogue between tumor cells, host cells, and inflammatory agents has become one of the epicenters of cancer investigations. Delineating the tumor margin is of paramount importance for tumor resection in the operating room, characterization of tumor progression, and/or remission under drug therapy in the clinic and to better understand the molecular pathways and drug targets involved in the tumor microenvironment [20].
Gross measurements using calipers are the primary method for preclinical measure of tumor size or volume. This method suffers from obvious inaccuracies and cannot be used longitudinally in many sites such as the brain. Because OCT benefits from relatively high soft tissue contrast, three-dimensional OCT can often be used to delineate tumor from host tissue at near cellular resolution. This use of OCT to probe tumor volume and boundaries is especially attractive in studies when OCT is also being used to probe another measure such as the tumor vasculature (Fig. 45.2). Here, because OCT structural images are obtained simultaneously with angiographic imaging, the overall imaging procedure does not need to be lengthened, and the additional information on tumor size and the ability to define intra- and extra-tumoral vessels is often valuable (Fig. 45.3). One drawback of OCT is that it is limited by its depth penetration; OCT can only image soft tissue up to a depth of 2 mm. Thus, larger tumors cannot be comprehensively imaged using OCT. However, OCT can probe more superficial segments of the tumor and often reveal growth/regression trends for this segment (Fig. 45.4).
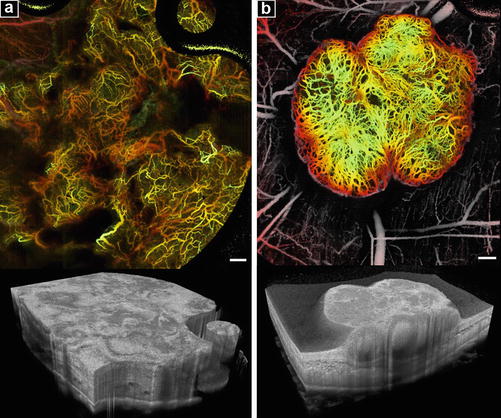
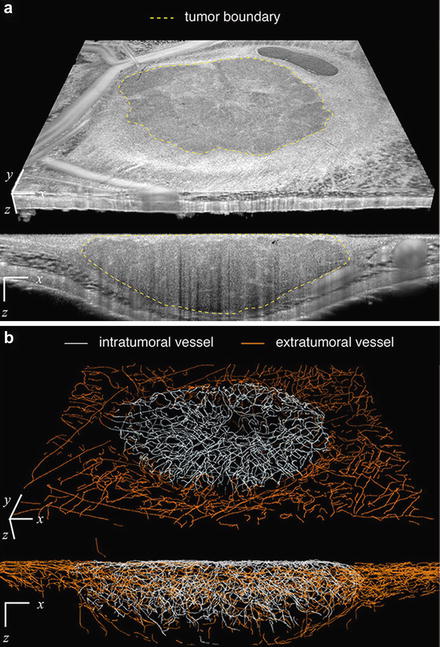
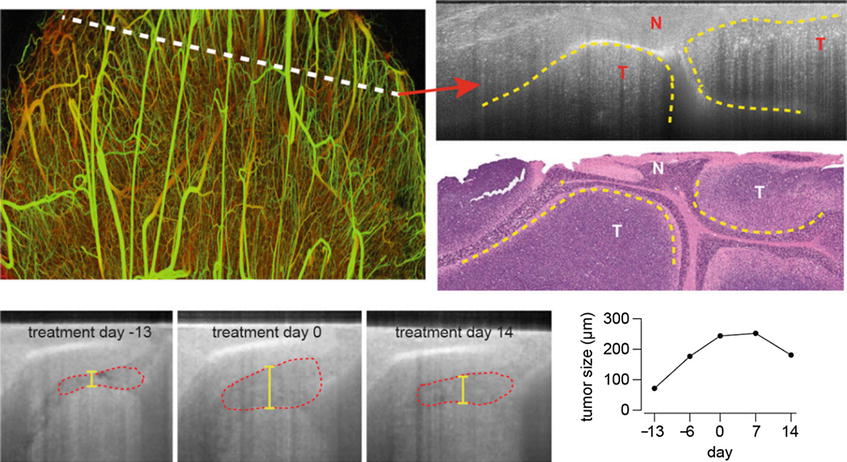
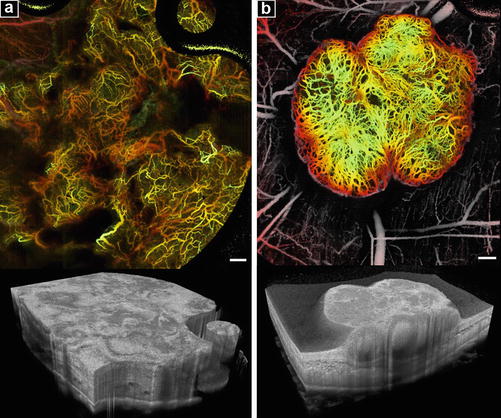
Fig. 45.2
OFDI angiography across tumor types and sites. (a) A human breast cancer cell line (MDA-MB-361HK) growing in the mammary fat pad window chamber model of a female SCID mouse. Large avascular regions are notable in the vascular image (top) and reflected in the topographically diffuse tumor microstructure (bottom). (b) Tumor vasculature of a human colorectal adenocarcinoma (LS174T) implanted in the dorsal skinfold chamber of a SCID mouse (top). Nonviable tissue is evident within the tumor nodules on cross section (lighter regions) in the tissue scattering intensity image (bottom). Scale bars 500 μm (Adopted from Ref. [6])
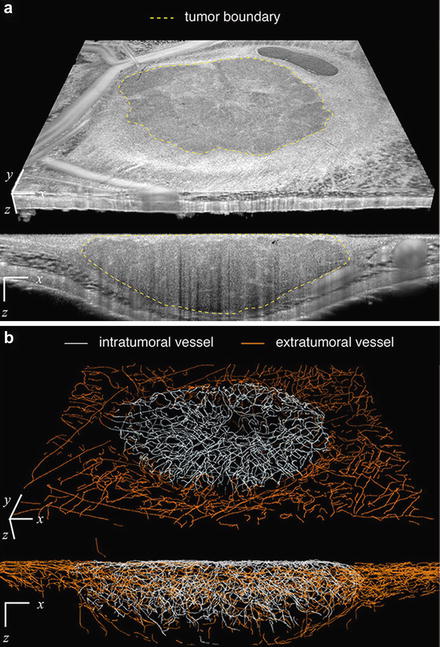
Fig. 45.3
Vascular tracing and structural correlation. (a) Microanatomical display showing tumor boundary definition in a three-dimensional tissue volume. (b) Skeletonized traced vessels differentiated between intratumoral and extratumoral for the tumor depicted in (a). Transverse extent in (a, b) 5 mm (x), 4.4 mm (y) (Adopted from Ref. [6])
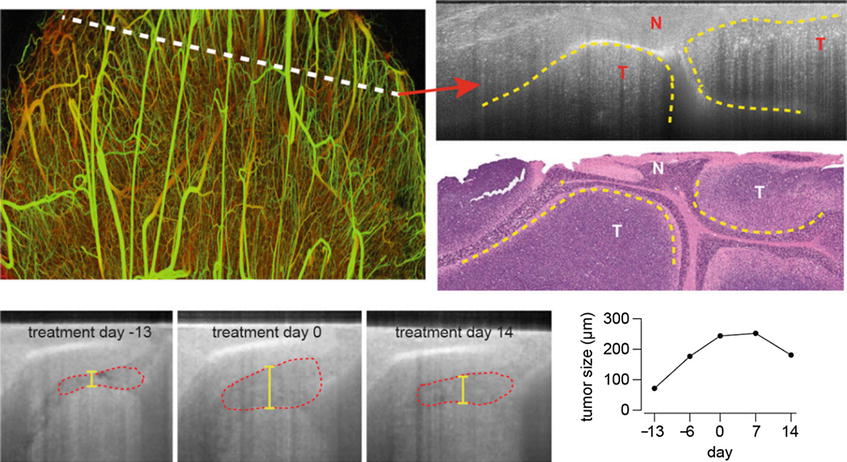
Fig. 45.4
Monitoring medulloblastoma growth using OCT. OCT images (top right) show distinct regions that can be correlated with normal (N) and tumor (T) tissues in histology (middle right). Longitudinal imaging shows growth and tumor regression with αPlGF treatment (bottom left). The red dotted line represents the tumor area observed with OCT, and the yellow line is the tumor diameter that was quantified during treatment (bottom right) (Reprinted from Ref. [7])
45.3 Tissue Viability Imaging
In addition to size, OCT signals can be used to differentiation between regions of viable and nonviable tissue within tumors. Tumors will commonly contain pockets of necrotic tissue due to insufficient perfusion. In addition, treatments often affect the tumor in a spatially heterogeneous manner, leading to differential viability. Viability imaging is typically done using micro-positron-emission tomography (μPET) using 18F-fluorodeoxyglucose (18F-FDG) as a radiographic contrast agent [2]. However, μPET has limited spatial resolution, usually greater than 1 mm. Fluorescence microscopy has also been used to monitor viability. Fluorescent deoxyglucose analogues have been used to study cell viability in cell culture as well as in vivo and may offer an alternative to PET imaging [38–41]. In addition, tumors expressing stable fluorescent proteins, such as the green fluorescent protein (GFP), will cease to produce the fluorescent protein upon cell death [42–44]. GFP has a half-life of 36 h, but its expression can be used as a crude measure of tissue viability. Both of these methods, however, are limited in their imaging field size and depth. In addition, they require exogenous agents to report viability.
It has been shown that OCT-detected tissue scattering magnitudes are dependent in some tumor models on the viability status of that tissue and that viable and nonviable tissues can be differentiated by analysis of the magnitude of associated OCT scattering signals [6, 45] (Fig. 45.5). Again, as with microstructural imaging, this viability contrast is derived directly from the microstructural images and can be combined with vascular and tumor margin measures. However, since the underlying cause of this increased scattering has not yet been elucidated, it is important to evaluate increased scattering in the context of other indications of viability. This is especially critical because increased scattering is not specific for viability changes.
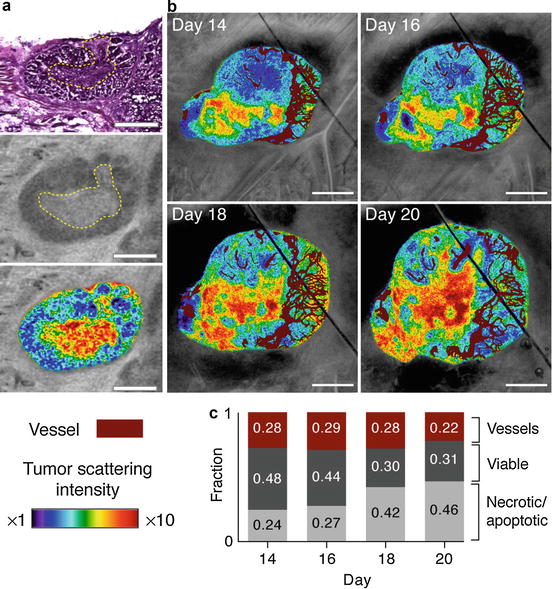
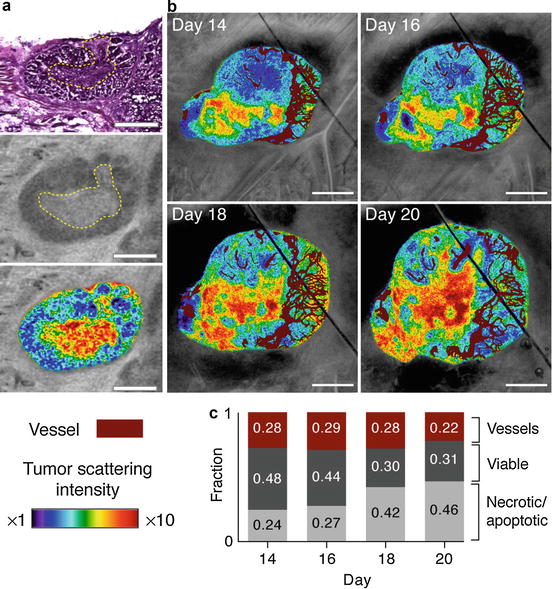
Fig. 45.5
Imaging tissue viability. (a) Comparison of standard hematoxylin and eosin staining (top) with OFDI (middle) reveals association of tissue necrosis with highly scattering regions. Viable and necrotic regions within the same tumor highlighted by color gradients indicating scattering intensity (lower). (b) Scattering properties correlated with the microvasculature during tumor progression illustrate the expansion of necrotic/apoptotic regions in areas with minimal vascular supply. Within the viable tissue, the mean distance to the nearest vessel was ∼65 μm throughout progression. (c) Quantitative analysis of tissue viability and vascular regions in vivo revealed an increase in the fraction of necrotic/apoptotic tissue from ∼24 % to ∼46 % during tumor progression. Scale bars (a) 500 μm; (b) 1.0 mm (Adopted from Ref. [6])
45.4 Lymphangiography
The lymphatic system is partly responsible for fluid homeostasis and protein transport in the body and plays an important role in the development of a tumor [46]. As a tumor grows locally, it begins to shed cells into the lymph. Some of these cells travel through the lymphatic vessels to new sites, thus making the lymphatic system a critical component of tumor metastasis. In addition, the growth of a tumor can cause lymphatic compression, which can affect convective drug transport [47]. Finally, the lymphatics are a critical component of the immune system. Thus, the functionality of the lymphatic vessels can determine the effectiveness of the immune response to cancer. For these reasons, the ability to image the lymphatics is important if we are to broadly understand the processes driving tumor development and treatment resistance.
Generally, intravital lymphangiography is more challenging than angiography. While contrast can be introduced intravenously for imaging the blood vessels, accessing the lymphatic system surrounding a tumor site is considerably more difficult. The most common method involves injecting a tracer locally into the tumor and waiting for the draining lymphatic vessels to take up the contrast [48]. However, lymphatic uptake can be heterogeneous, so this technique often only allows imaging of a partial lymphatic network. Furthermore, local injection of contrast can disrupt the tumor microenvironment, including the lymph.
Lymphangiography with OCT is possible due to the optical transparency of lymph fluid [6, 49]. The lymphatic fluid does not significantly scatter incident light, which is in stark contrast to the surrounding highly scattering soft tissue. Thus, in an OCT image, the lymphatic vessels appear hypoechoic when filled with lymph (Fig. 45.6). In addition, the lymphatic vessels have a unique valve and lymphangion morphology, allowing easy identification of lymphatic structures. A notable limitation of this method is that the lymphatic vessels are not always expanded and filled with fluid. This can sometimes make comprehensive visualization of the lymphatic networks difficult. However, this limitation may be overcome with the use of higher-resolution OCT systems. OCT lymphangiography in the study of cancer has been performed in both ear models and dorsal skinfold chamber models. In recent work, OCT lymphangiography was used to track single lymphatic vessels longitudinally, where it demonstrated the presence of lymphatic hyperplasia in the peri-tumor environment.
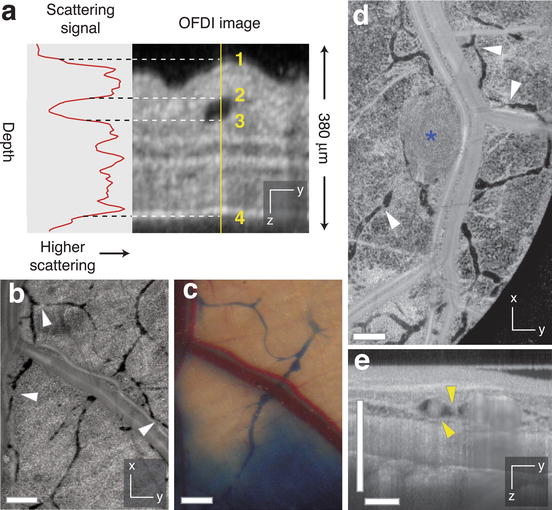
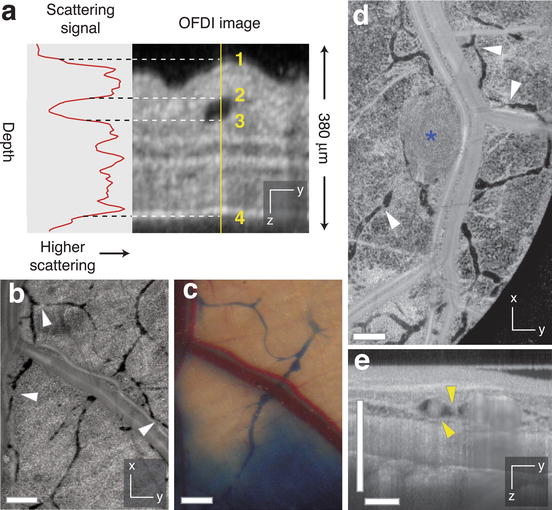
Fig. 45.6
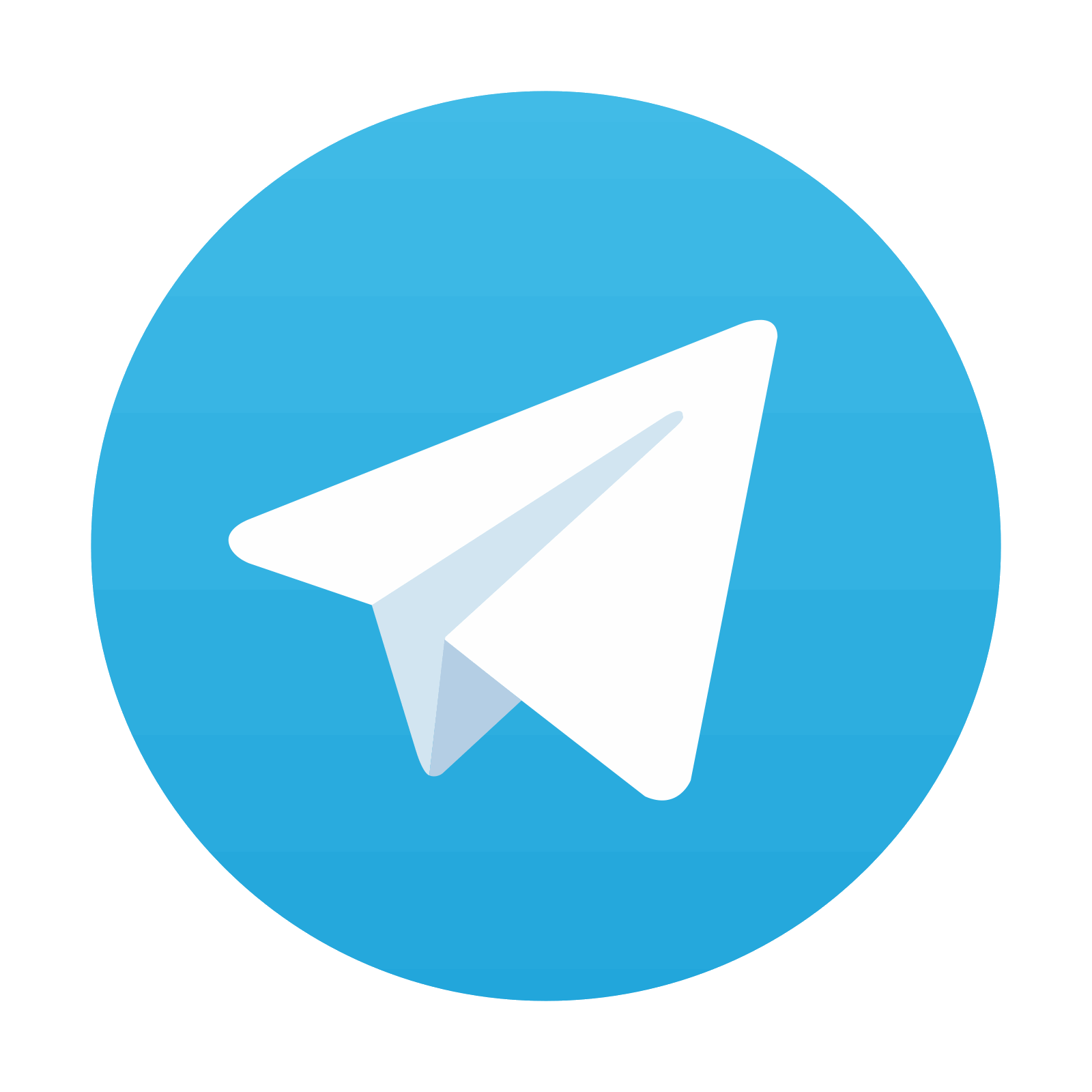
Contrast-free lymphangiography using OCT. (a) The scattering signal along a single depth scan within an OFDI image of a mouse ear, showing the reduced scattering between the upper (2) and lower (3) boundaries of a patent lymphatic vessel. Scattering within the vessel is similar to background levels above the upper surface of the ear (1) or below the lower surface (4). (b, c) In addition to the lymphatic vessels revealed by traditional cutaneous injection of Evan’s blue dye imaged by wide-field transillumination with a CCD camera (c), OCT lymphangiography (b) was able to detect numerous additional vessels in the normal dorsal skin and resolve the lymphatic valves found between individual lymphangions (white arrowhead). (d) OCT lymphangiography showing hyperplastic lymphatics associated with HSTS26T tumor (blue asterisk). (e) Cross-sectional presentations of OFDI lymphangiography showing cellular masses in a lymphatic vessel (yellow arrowhead) located near the tumor in (d). Scale bars, 500 μm (Adopted from Ref. [6])
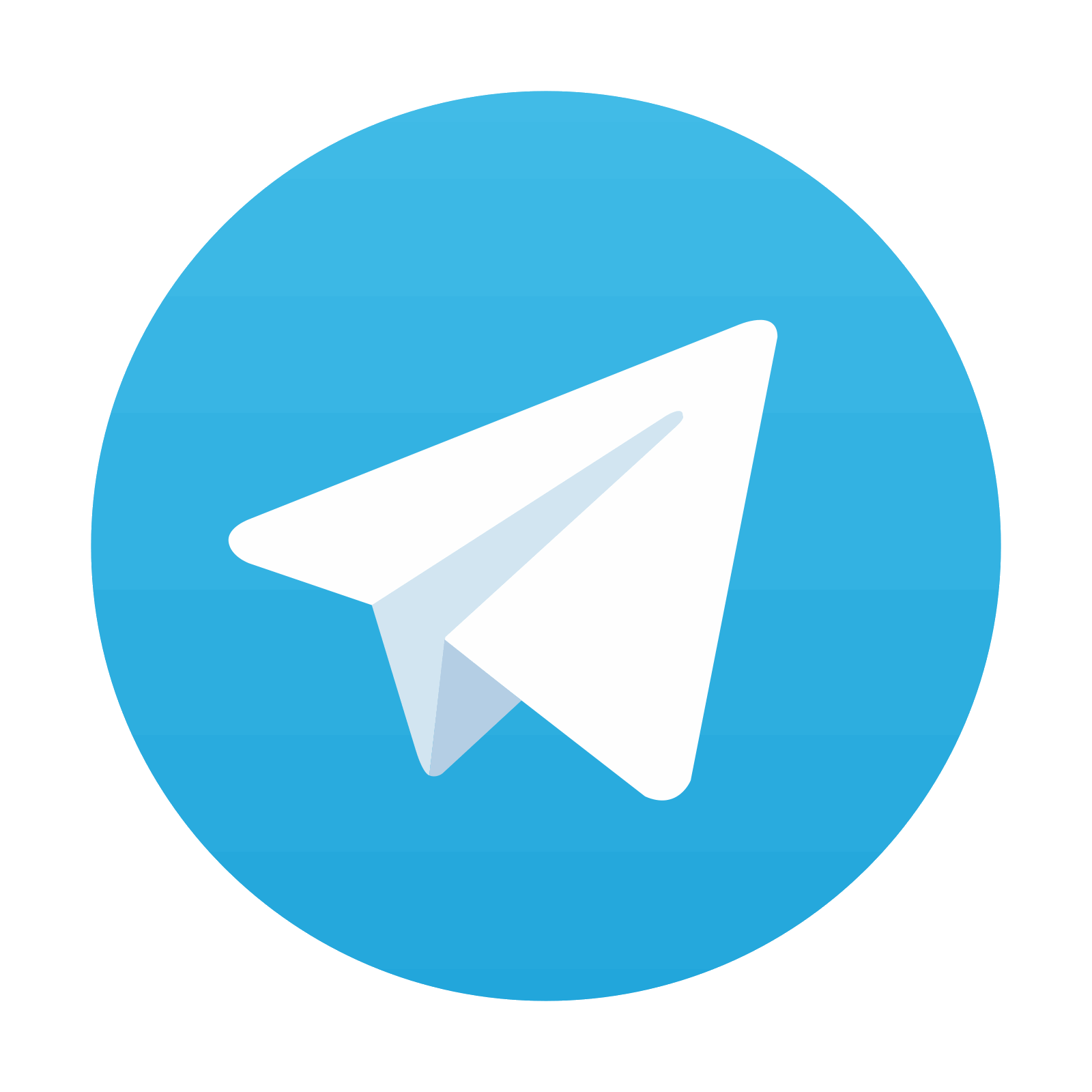
Stay updated, free articles. Join our Telegram channel
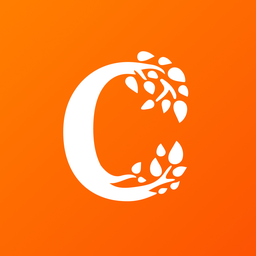
Full access? Get Clinical Tree
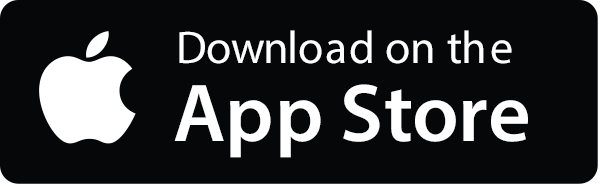
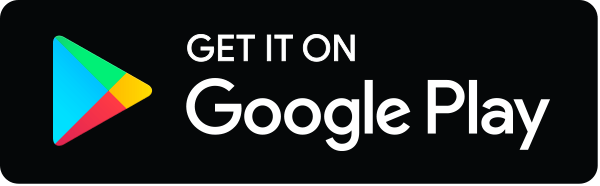
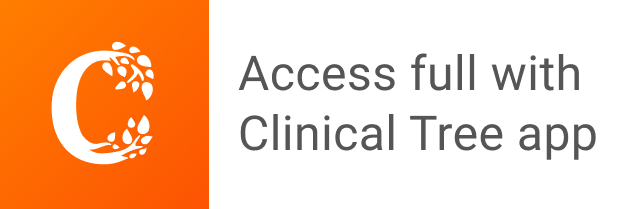