Disorder
Sensitivity (%)
Specificity (%)
Specialized intestinal metaplasia [42]
81
57–66
Dysplasia [43]
68
82
HGD/carcinoma [44]
83
75
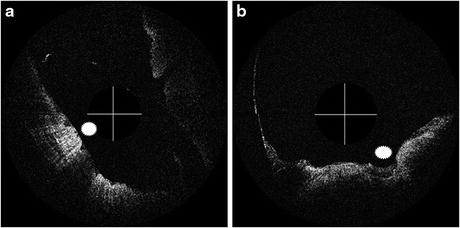
Fig. 67.1
Endoscopic TD-OCT images from a study in dysplasia diagnosis [43]. (a) LGD (area marked by sunburst). (b) HGD (area marked by sunburst). Two criteria for diagnosing dysplasia were selected: reduced light scattering and loss of tissue architecture. Solid lines are each 2 mm in length
The early EOCT studies above utilized the first-generation EOCT system built in a time-domain configuration. Those early EOCT imaging systems had two major disadvantages: slow imaging speed and small field of view. Therefore, the surface area of mucosa interrogated in any given image was exceedingly small (typically about 6 mm image width by 25 μm slice thickness), making such systems unsuitable for interrogation of large mucosal regions.
One of the latest advances in OCT technology was ultrafast imaging speed, which led to the advent of the second-generation EOCT system. These new EOCT systems benefit from ultrahigh A-scan acquisition rate of Fourier-domain OCT. Miniature catheter probes with centering balloons and more than 10 mm working distance are another key technology which allows for scanning a lumen with a large diameter. New technologies make comprehensive esophageal screening and surveillance possible [48–53]. It was recognized that EOCT imaging could be extended to cover a much larger area of mucosa than that sampled by the conventional biopsy forceps. The aim of eliminating sampling error in current BE diagnosis procedures has motivated the development the second-generation of EOCT for volumetric esophageal imaging.
The feasibility of this second-generation EOCT was first demonstrated in swine esophagus in vivo [49, 51, 52]. More than three centimeters of esophagus was visualized up to the muscularis externa (smooth muscle) with unparalleled resolution in minutes. 3D reconstruction allows the longitudinal and en face views, in addition to the conventional cross-sectional views. The entire circumference can be scanned with rotary catheter probes when the tissue is expanded with cylindrical balloons. The vasculature in the muscularis mucosa was clearly visualized by extracting the Doppler shift from OCT signal. The impact of the balloon compressing the tissue was questioned [54]. A double-balloon catheter was proposed as an alternative design so that the mucosa to be imaged was not in contact with balloons [52]. A catheter probe without the centering balloon was also demonstrated [55]. This probe achieved higher lateral resolution due to short working distance, with the tradeoff of imaging only a portion of the circumference. Also demonstrated was the feasibility of producing laser ablation marks in live swine using the OCT catheter probe [56]. The ablation site was visualized both in EOCT images and biopsy slides. This technology may be potentially utilized in a clinical setting to precisely correlate EOCT with pathology.
Pilot clinical trials have also been reported [50, 55, 57]. The image acquisition required only minutes to accomplish. No complications have been reported resulting from the imaging procedure. A variety of microscopic features were observed that were consistent with histopathologic findings, including squamous mucosa, intestinal metaplasia with and without dysplasia, Barrett’s glands, and esophageal erosion. 3D EOCT has also been investigated as a comprehensive imaging tool to detect residual Barrett’s glands after ablation treatments [55, 58]. It may benefit the ablation procedure by reducing the recurrence of Barrett’s esophagus and the need for secondary ablation and potentially further increase the complete eradication rate of intestinal metaplasia.
67.2.2 Colon
The early clinical EOCT studies in colonoscopy, similar to early esophageal studies, were limited by the imaging speed and field of view of the first-generation EOCT systems. These studies investigated the backscattering profile of single cross-sectional images. Shen et al. discovered that EOCT demonstrated disruption of the wall structure in the majority (90 %) of patients with Crohn’s disease, whereas the disrupted structure was present in significantly fewer cases (16.7 %) of ulcerative colitis [59]. Consolo et al. demonstrated that mucosal backscattering alteration was an effective feature to detect inflammatory bowel disease in colon with sensitivity of 100 % and specificity of 78 % [60]. 3D endomicroscopy of a large area in the colonic wall with high resolution has recently been demonstrated with the advent of the Fourier-domain EOCT technology, enabling the visualization of single crypts [61]. One of the potential clinical applications was to identify ACF, a possible precursor of precancerous polyps. Qi et al. developed an automated algorithm for colonic crypt segmentation and morphologic feature characterization [62]. The algorithm was applied to 3D OCT images obtained from excised human colonic tissues, which demonstrated the potential to differentiate ACF from normal crypts.
67.3 EOCT Key Technologies
State-of-the-art EOCT research focuses substantially on cancer precursor detection in large areas within the GI tract in a clinical setting. Visualizing the tubular organs requires several key technologies. Most of these technologies are developed in the sample arm, making the common OCT configuration usable in the GI tract. The image acquisition has to be fast because the EOCT procedure is expected to be accomplished within minutes. The imaging probe has to be reasonably small and flexible which allows for easy deployment in the lumen of tubular organs. The optical and mechanical design of the catheter directs the probing beam so that the large mucosal area of the tubular organ can be scanned. On the software side, motion artifact correction is required because physiological motion causes artifacts with similar characteristics as nonuniform rotary distortion (NURD). This causes significant misalignment along the longitudinal direction, and the distorted images may diminish the utility of 3D EOCT. Computer-aided diagnosis (CAD) algorithms are also potentially important to assist the challenging tasks of discerning dysplasia and other abnormalities, by providing objective and quantitative interpretation, reducing inter- and intra-observer variability, and speeding image interpretation. 3D EOCT procedures may yield thousands of images per patient. Analysis of these data by the unaided human reader will not be practical.
67.3.1 EOCT System
Currently, swept-source OCT is more often implemented for endoscopic applications than spectral-domain OCT. Currently, commercially available swept laser sources have higher line-scan rates than that of line-scan cameras used for spectrometers for the wavelength of 1,310 nm, which is commonly used in dense tissue imaging. The sensitivity falloff range in a swept-source system is usually more than 6 mm, compared to less than 2 mm in spectral-domain OCT. The complex conjugate artifact can be more easily resolved, e.g., with frequency shifting [63] or other technologies. Swept-source OCT can also be built with all fiber-optic components, without the need to design and maintain a high-speed spectrometer. While SDOCT may potentially have advantages over SSOCT in terms of axial resolution and phase stability, and high-quality EOCT has been implemented in both SSOCT and SDOCT configurations [31, 64, 65], in the foreseeable future, swept-source OCT will likely remain the preferred configuration for gastroenterologic applications.
67.3.2 Miniature Probe
The fiber-optic probe guides the light from the EOCT system to the internal organ, focuses the beam onto the mucosa of the luminal wall, and collects the image-bearing backscattered light. A small outer diameter and short rigid parts are preferred for ease of use, especially when the probe in intended to be introduced through the accessory channel of an endoscope. The side-viewing design is most commonly used. When driven in a helical scanning pattern, the probing beam can scan the inner wall of the tubular organs. The probe may be used with or without a centering balloon, depending on the application and the needed optical performance. Balloon-based probes have long working distances, corresponding to the diameter of the balloon. The probe beam passes through the balloon and is maintained in focus on the entire circumference. They are mostly used for large, straight tubular organs, e.g., esophagus. For a typical (Gaussian) beam, the consequence of a long working distance is a larger beam waist, in other words, lower lateral resolution. Probes without balloons typically have a working distance barely longer than the outer protecting catheter sheath and may therefore be focused to a sharper spot. They are placed directly adjacent to the tissue when imaging, and if the organ has a large diameter, only a portion of the circumference is scanned. Short working distance probes are the better choice for imaging pancreatic/bile duct, which have small lumens, and colon if it is to be imaged in a targeted, rather than comprehensive way.
Figure 67.2a shows an example of a probe design for a balloon catheter [52]. A single-mode fiber was fixed in a ferrule followed by a glass spacer to expand the beam before entering a GRIN lens. A separate cylinder rod lens was attached after the GRIN lens to correct the astigmatism induced by the protecting sheath. All optical components have polished surfaces angled at 8° to prevent back reflection. The length of the spacer may be selected to result in the desired working distance of the lens group. The lens group and ferrule were assembled in a metal housing. A gold mirror was glued at the tip of the metal housing to deflect the beam by 80° relative the optical axis. The overall numerical aperture of the probe is 0.024. The total outer diameter of the fiber probe, including the metal housing, is 1.7 mm. The rigid tip (medal housing) is about 2 cm long. Figure 67.2b shows the size of an assembled probe without the metal housing. Figure 67.2c shows the lateral beam profile at a 9 mm working distance, as measured by a beam analyzer. The spot had a minimal ellipticity of 0.98 with a FWHM diameter of 33 μm.
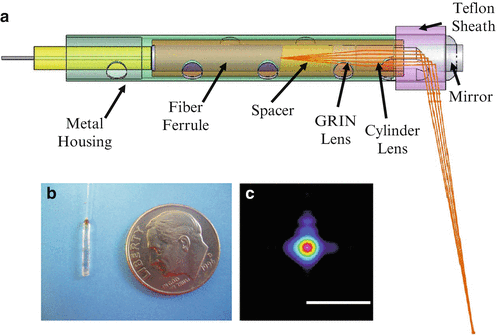
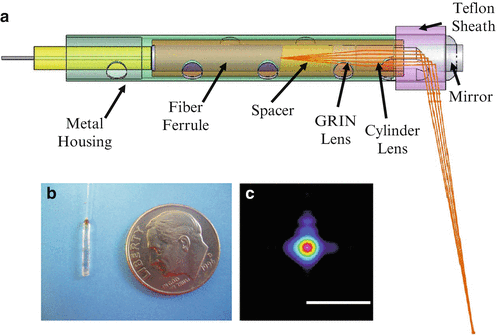
Fig. 67.2
(a) The mechanical model of the probe design. (b) An assembled fiber-optic probe without metal housing. (c) Beam profile measured by a beam analyzer. (Bars: 100 μm)
In order to perform the helical pattern scanning, a rotary motor and a translational motor were utilized to drive the proximal end of a flexible shaft. The metal housing of the probe was attached to the distal end of the flexible shaft. The flexible shaft can mostly prevent nonuniform rotary distortion (NURD). To further suppress NURD, The position encoder in the rotary motor was used to trigger the OCT A-scan acquisition at equal angular increments.
67.3.3 Balloon Catheter
BA balloon centers a long working distance probe to maintain focus and to stabilize the probe to suppress motion artifacts. The conventional balloon design uses a single balloon, with its two necks attached to the outer sheath using biocompatible epoxy. The imaging probe is placed within the balloon, and the probe beam passes through the balloon and is focused into the adjacent mucosa. It has been shown that catheter pressure on colonic mucosa significantly alters the tissue appearance [66]. This raises the question of whether balloon pressure or contact compresses the mucosa and affects OCT image features that are diagnostic of dysplasia within BE.
Double balloons were proposed as an alternative design [52]. Figure 67.3a illustrates the double-balloon design that allows two imaging schemes to be employed (with and without balloon-tissue contact) to investigate the influence of pressure. For imaging with no balloon-tissue contact, the images were obtained in the gap between the two balloons (labeled beam position (1)). The fiber probe could also be placed within the distal balloon (labeled beam position (2)) to acquire images through the balloon in the conventional way. The length of the gap was well controlled in order to limit the extent of lumen contraction between the balloons and keep the tissue within the axial imaging range. Holes were made in the outer sheath within the balloons so that they were inflated and deflated from the proximal end of the catheter. The outer sheath had an outer diameter of 2.4 mm. The small diameter allowed the catheter to be inserted through the accessory channel of the endoscope (Fig. 67.3b). The deployment and operation of the catheter probe were observed from the camera of the endoscope, so that images were acquired from the esophageal segments of interest (Fig. 67.3c). Figure 67.3d represents a swine esophageal image in anatomic (cross-sectional) view. The diameters of the imaged esophagi were around 18 mm, but in order to visualize the tissue structure, the images are displayed with a diameter of about 3 mm.
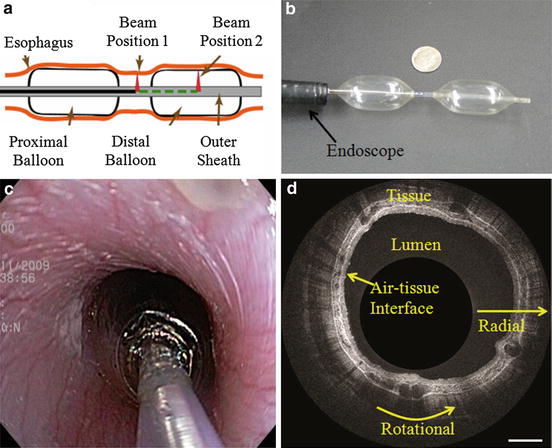
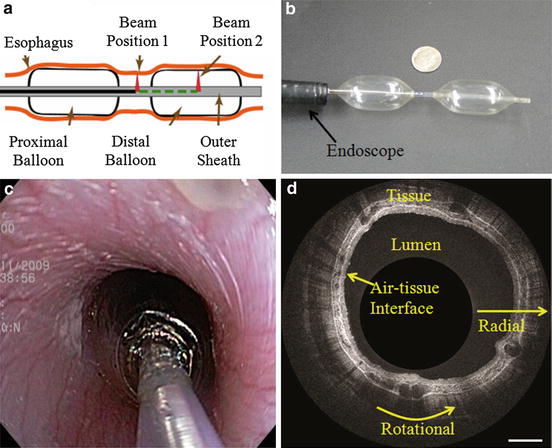
Fig. 67.3
(a) Schematic of the double balloon design. The beam can be located between two balloons for imaging without balloon-tissue contact (solid black line) or in a balloon for imaging with contact (dashed green line). (b) The double-balloon catheter (compared with a dime) inserted through the GI endoscope and inflated. (c) The deployment of the balloon catheter in swine esophageal observed from the camera of the endoscope. (d) A cross-sectional image of swine esophagus in anatomic view (Bar: 1 mm)
67.3.4 Motion Artifact Correction
In previous sections, several methods have been introduced for the purpose of motion artifact suppression, i.e., triggering with the encoder in the rotary motor, rotating the probe with a flexible shaft, and stabilizing it with the balloon. However, when imaging living subjects, motion artifact may not be completely suppressed. A study has shown that the motion artifact can be caused by physiological motion, e.g., respiration and heart beating [57]. Figure 67.4a demonstrates an example of motion artifact in the swine esophagus in vivo. Cross-sectional images were acquired for one minute without pulling the probe and stacked in the order of time (time axis). All of the above motion suppression methods were applied. Ideally without motion, these images should be identical. However, the time-radial plane (Fig. 67.4c) illustrates how the reconstruction is substantially distorted radially. Figure 67.4b is an en face plane extracted from the same volume within the layer of the muscularis mucosa (approximately 300 μm from the mucosal surface). In this view, rotational distortion is clearly observed. Motion artifact along the longitudinal direction (perpendicular to the cross-section) is also prominent. However it cannot be clearly visualized without additional imaging mechanism (i.e., Doppler shift detection [67]). Here, motion artifacts refer to the radial and rotational components if not otherwise specified.
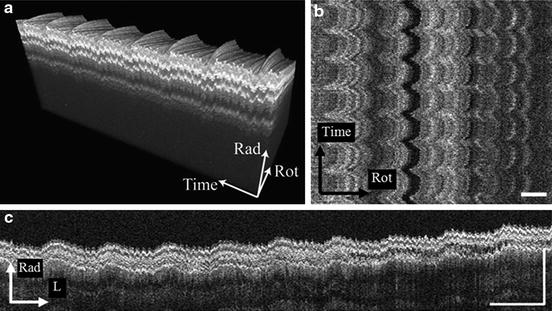
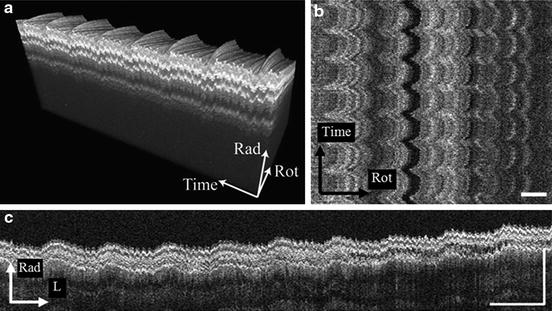
Fig. 67.4
(a) 3D reconstruction of swine esophageal images obtained without pulling the probe. The radial-rotational plane should not change over time if there was no motion artifact. (b) The en face view of the plane 300 μm deep in (a) shows rotational motion. (c) The radial-longitudinal view from the 3D reconstruction shows the radial motion (Rad radial, Rot rotational, L longitudinal. Bar: 1 mm)
Kang et al. proposed an automatic image registration method to suppress motion artifact during esophageal imaging [57]. Registration started with the detection of esophageal lumen, assuming the air-tissue interface had the highest radial gradient. The detection was formulated as a global optimization problem, which sought a contiguous curve with only one pixel from each A-scan. The summation of the radial gradient value on the curve was a global maximum among all the possible curves. To correct for radial motion, the detected lumen surface was aligned to a circle by translating each A-scan in the radial direction. Local box matching (LBM) was then applied to estimate the rotational motion. LBM matched large regions of interest (ROI) in each image to the previous image by cross-correlation and therefore estimated the bulk rotational motion artifact of the ROIs. Each ROI consisted of a few hundred of A-scans. The rotational position of each individual A-scan was then finely adjusted by considering the bulk motion of the adjacent ROIs in the same cross-sectional image. This method can be categorized as a template update method in the field of computer vision [68]. In this case, the previous cross-sectional image was the template. Accumulated registration error occurred because LBM did not distinguish tissue motion from the actual variation in the anatomical structure. To visualize the natural tissue structure, the slowly varying components in the motion trace were preserved during the final image reconstruction.
67.3.5 CAD
CAD algorithms have been proposed to analyze colonic crypt morphological patterns [69] and to classify dysplasia in BE [45, 46]. CAD algorithms usually consist of steps including region of interest (ROI) segmentation, feature extraction and selection, and finally classification. It is critical to first identify appropriate and quantifiable target features for computer processing before CAD algorithm development. The straightforward approach is to adopt and quantify the diagnostic criteria established by human expert readers.
EOCT visualizes the 3D structure of the colonic mucosa, which is one of the advantages over other competing colonoscopic imaging technologies, such as high magnification chromoendoscopy. The crypts within aberrant crypt foci (ACF) have larger lumens and are oriented less parallel to each other in three dimension than normal crypts are [70]. Qi et al. proposed to characterize the crypt morphology using 3D image processing to quantify these 3D morphological features [69]. The process was demonstrated using freshly resected segments of colon from patients undergoing surgery to remove cancer. Crypts were first segmented on each en face section of the 3D dataset. Features, such as area of the lumen and density of crypts (counts per square millimeter), were calculated. The centroids of each segmented crypt were then connected to represent the crypt morphological skeleton. Straightness (i.e., a skeletons’ spatial linearity) and parallelism (i.e., the similarity of the skeletons’ spatial orientation) were computed. All the features were quantified for ACF, normal tissue, and normal-appearing tissue adjacent to cancer (NA). Different feature values were observed between ACF and normal/NA tissue, which may potentially be useful for differentiating abnormal crypts from data acquired in vivo.
CAD algorithms have also been developed to potentially assist in diagnosis of dysplasia in BE [45, 46]. The transformation from normal to dysplastic mucosa in BE includes the size, shape, and density of epithelial nuclei, which affects the tissue attention and backscattering properties. Loss of structure associated with normal histological organization (i.e., homogeneity, layering) has been observed in dysplastic tissue [37, 43, 71]. Some of these previous observations were quantified, such as backscattering coefficient and intensity-based texture parameters, for CAD algorithms. New features, such as the stripe pattern associated with surface topology, were also proposed for CAD algorithms [72, 73]. A stripe pattern was often observed in non-dysplastic tissue and seldom observed in dysplastic tissue. This pattern was quantified by the stripe density and orientation. This method was demonstrated using clinical EOCT images from patients undergoing regularly scheduled BE surveillance, where diagnoses were confirmed by biopsy. The receiver operating characteristic (ROC) curve of each feature was calculated to select those with best classification accuracy. Principle component analysis was applied to reduce feature redundancy and increase algorithm robustness. Only a few principle components that accounted for the most variance in the feature space were selected as input for the multivariate classification algorithms. Multiple classification methods were compared. In this case, decision tree combined with bootstrapping yielded best classification accuracy. Bootstrapping consisted of construction of multiple classification training sets, each using a randomly selected subset of the EOCT training images. Each training set generated a decision tree, and then each test image was classified by majority voting of those decision trees [45, 46].
67.4 Recent EOCT Imaging Studies
It has been shown that endoscopic OCT (EOCT) can obtain interpretable images of gastrointestinal (GI) mucosal microstructure, differentiate GI mucosal types, and detect abnormality ever since the first-generation time-domain systems [30–40, 59, 74–76]. State-of-the-art EOCT studies in GI tract utilize the second-generation Fourier-domain systems. The aims have been extended to investigate the clinical impact of ultrafast image acquisition, eliminate sampling error, and visualize new diagnostic features, such as buried glands in BE and crypt patterns in colon in vivo.
67.4.1 Animal Studies
Swine esophagus is a commonly used animal model for in vivo studies to demonstrate feasibility of new technology. Kang et al. reported a spectral-domain EOCT system together with double-balloon catheter probe [52]. The fiber probe was rotated at 10 rev/s and pulled back at 2 cm per minute. One centimeter to three centimeter segments of esophagus were imaged. Each cross-sectional frame consisted of 4,700 A-scans. The image resolution was 9 μm, 33 μm, and 33 μm in radial, rotational, and longitudinal directions, respectively. The image voxel size was 4 μm, 11 μm, and 33 μm in the three directions. Swine were sedated, intubated, ventilated, and maintained under anesthesia for the duration of the procedure. During the imaging study, video endoscopy was used to guide the probe deployment and through the endoscope accessory channel and balloon inflation.
Both double-balloon and single-balloon images were obtained from the same esophageal segment (Fig. 67.5) in order to evaluate relative image quality and the effects of the pressure of the balloon on the OCT appearance of the mucosa. Figure 67.5a is a representative cross-sectional image of the swine esophagus without balloon-tissue contact. The same radially compressed visualization method used in Fig. 67.3d is applied here. Tissue layers and structures can be clearly observed, including the squamous epithelium, lamina propria, muscularis mucosa, submucosa, muscularis propria, and blood vessels in the muscularis mucosa. The imaging depth exceeds 1 mm. Figure 67.5b shows a 3D reconstruction visualizing a 17 mm long segment obtained within 50 s, using the double-balloon scheme. Figure 67.5c, d show a representative cross-sectional image and the corresponding 3D reconstruction obtained with the single-balloon scheme (10 mm segment obtained within 30 s). The typical layers of the esophagus can also be clearly recognized. The imaging depth in the single-balloon images is greater than that of the double balloon, with multiple layers of muscularis propria visible. However, detailed structure in the muscularis mucosa, especially blood vessels, is more clearly observed in the double-balloon images. The glandular structure and the blood vessels are barely recognized in the single-balloon image. The natural mucosal surface topology is apparent in the double-balloon image, whereas the mucosal surface was compressed and smoothed by the balloon in the single-balloon image. The balloon-flattened surface resulted in more stable illumination and therefore more uniform image brightness. Also, the tissue motion artifact was less significant with single-balloon imaging due to the balloon support. These observations suggest the need to further investigate the advantages and disadvantages of balloon-tissue contact and pressure, how tissue features of diagnostic significance in BE are altered, and how the changes affect detection of dysplasia in BE.
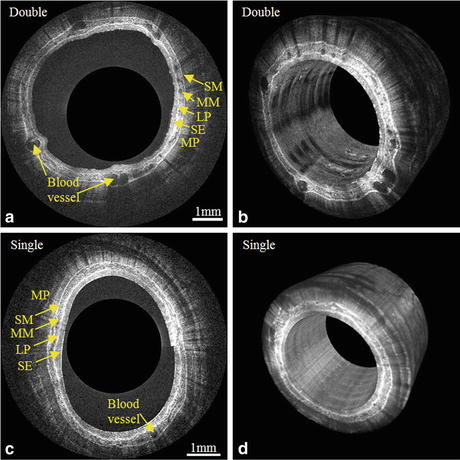
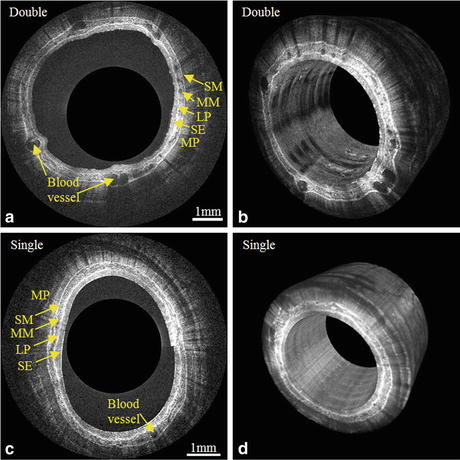
Fig. 67.5
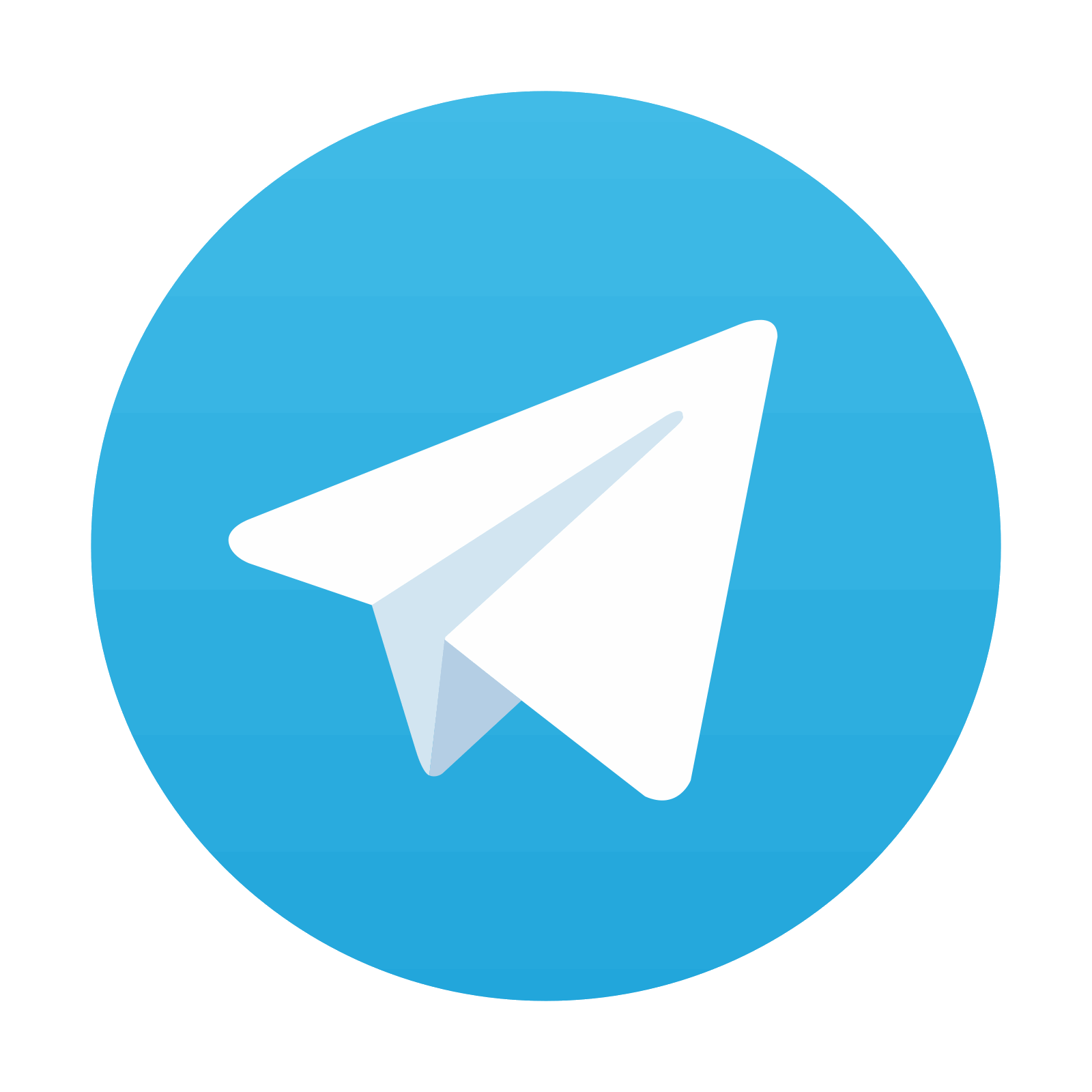
(a) A representative cross-sectional image of a swine esophagus with the double-balloon imaging scheme. The layered structure that can be observed includes the squamous epithelium (SE), lamina propria (LP), muscularis mucosa (MM), submucosa (SM), and muscularis propria (MP). (b) 3D reconstruction of a 17 mm long section of swine esophagus obtained with the double-balloon imaging scheme. (c) A representative cross-sectional image of the single-balloon imaging scheme. (d) 3D reconstruction of 10 mm long segment with single-balloon imaging scheme
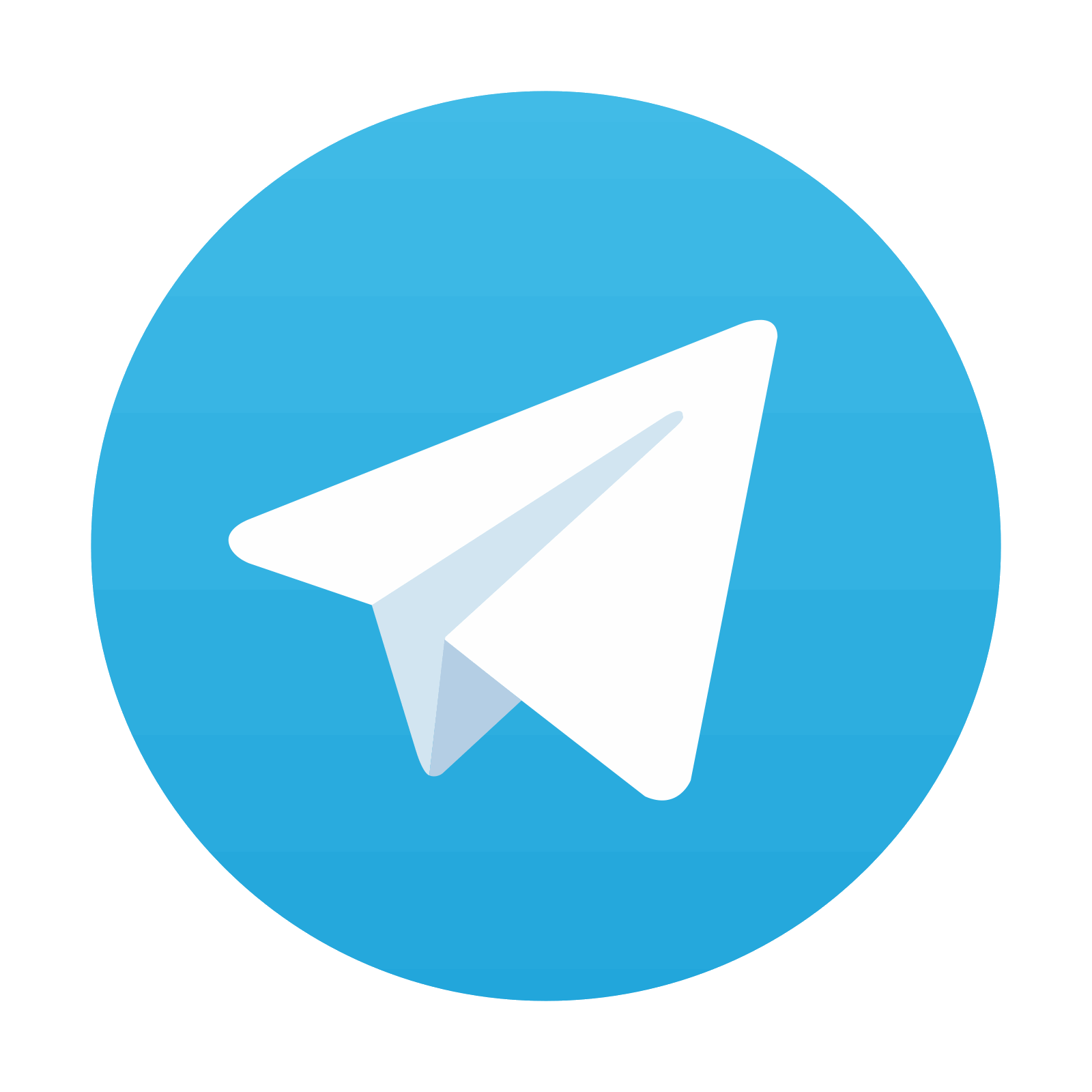
Stay updated, free articles. Join our Telegram channel
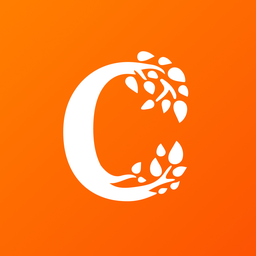
Full access? Get Clinical Tree
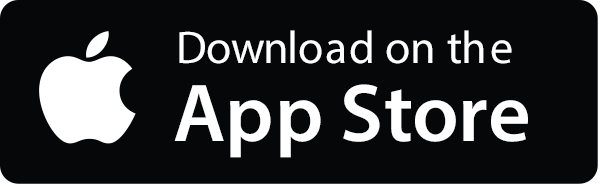
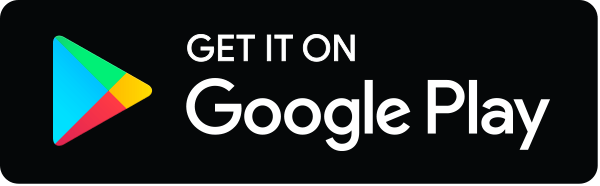
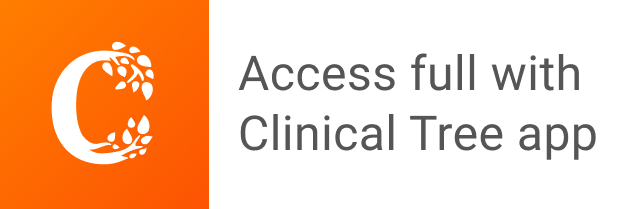