Fig. 3.1
Overview of neurotrophin expression and effects of neurotrophin loss. Two neurotrophins, Bdnf and Ntf3, are expressed in the developing organ of Corti. Bdnf is nearly exclusively found in hair cells and Ntf3 mostly in supporting cells but also IHCs (a). Ntf3 is also expressed in the developing cochlear nuclei (CN). Two types of spiral ganglion neurons (type I, type II) project to IHCs and OHCs, respectively, and coexpress the two neurotrophin receptors, Ntrk2 and Ntrk3. b Loss of either both neurotrophins or both neurotrophin receptors results in complete loss of all spiral ganglion neurons at birth, reduction in cochlear nucleus size, and, after several months, loss of OHCs followed by loss of IHCs
More recently it has become clear that the original formulation of the neurotrophic theory, meant to explain how neurotrophins regulate the viability of properly connected neurons for normal function of the adult system, was too simple. Some receptors play dual roles as “dependence receptors”: They are death promoting, pro-apoptotic without neurotrophins, but prevent death with neurotrophins (Taylor et al., 2012; Dekkers et al., 2013). Moreover, even deletion of two neurotrophins has only a very limited effect on the central nervous system (CNS) (Fritzsch et al., 1997c), indicating that perhaps CNS cell death is not regulated the same way as in the peripheral nervous system (PNS) (Dekkers & Barde, 2013). These new insights suggest not only that the neurotrophic theory may have limited value for the CNS but also that even in the developing PNS many examples do not fit well to the theory. As will be apparent in the narrative that follows, ear development superficially fits the neurotrophic theory. Closer examination reveals, however, that neither correction of misguided afferents through elimination of parental neurons or pruning, nor an easy numerical correlation of afferents to target hair cells, occurs in the ear.
The molecular basis supporting the viability of sensory neurons by the developing sensory epithelia of the inner ear has been clarified through targeted mutational analysis in mice. Genetic ablation of two neurotrophic factor genes or their two receptors (Fig. 3.1) rapidly kills all sensory neurons during embryonic development (Ernfors et al., 1995; Fritzsch et al., 1997c). In contrast to molecular understanding of neuronal dependency on factors released by sensory epithelia, progress in understanding the molecular basis of cochlear nuclei dependency on afferents has been limited. It has been suggested that cochlear nucleus neurons depend on either transmitter release and/or co-release of yet to be determined substance(s) from spiral ganglion neurons for survival (Rubel & Fritzsch, 2002). In addition, neurons of one vestibular nucleus of birds (Shao et al., 2009), but not of several other vestibular nuclei in birds and mammals, critically depend on inner ear afferents for survival. Even those vestibular and cochlear nuclei that show induced cell death on denervation lose fewer than 50 % of all neurons, comparable to other developing systems (Oppenheim, 1991). This limited effect of denervation on central nuclei contrasts sharply with loss of neurotrophins on ganglion neurons: all vestibular and spiral ganglion neurons degenerate after genetically engineered removal of both neurotrophin factors or receptors (Yang et al., 2011).
To complicate matters further, mice genetically engineered to be unable to release synaptic or dense core vesicles (Fig. 3.2) through the mutation of proteins needed for vesicle docking show normal assembly and synapse formation in the absence of any vesicular release (Verhage et al., 2000; Varoqueaux et al., 2002). Importantly, dense core vesicles are believed to store and release neurotrophins (Kuczewski et al., 2009; van de Bospoort et al., 2012), in particular pro-forms (Dieni et al., 2012). In vesicular docking defected mutants, there is a phase of enhanced neurodegeneration (Verhage et al., 2000), and neurotrophins can offset the neuronal loss for some time (Heeroma et al., 2004), arguing that the cell death in these mutant mice may be caused by other intracellular functions such as intracellular vesicular fusion to Golgi cisternae (Ma et al., 2013). This conclusion is further supported through data in related mutant mice that have no synaptic transmitter release, show no enhanced cell death, but do show normal development of the brain until birth as well as normal synaptogenesis in postnatal brain tissue culture (Varoqueaux et al., 2002). Combined, the data on vesicular docking mutants strongly support the notion that during embryonic development there is little evidence for activity mediated fine-tuning of connections facilitated by vesicular release. Deletion of vesicular docking proteins only in sensory neurons is needed to verify that connection development can progress without vesicle release. Such mutant mice should demonstrate postnatal neuronal loss comparable to cochlear ablation (Harris & Rubel, 2006). Depending on the outcome of such an experiment, it might be necessary to reconcile possibly conflicting data with the prevailing view and interpretation in the current literature on the role of activity dependency mediated by vesicle release (Marrs & Spirou, 2012). In summary, neuronal activity of cochlear afferents leading to glutamate release, possibly paired with co-release of dense core vesicles containing an unknown factor(s), may be the basis of afferent support of cochlear nuclei (Rubel & Fritzsch, 2002).
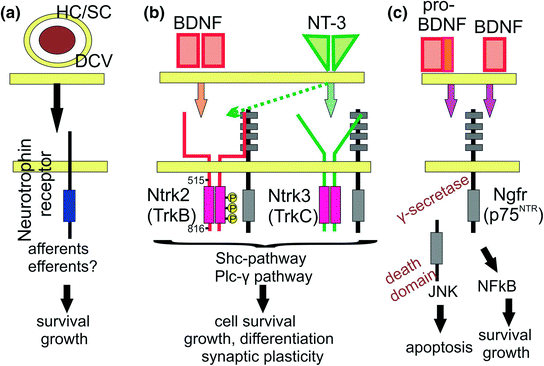
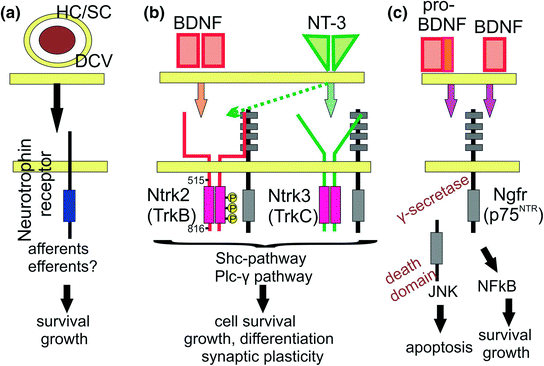
Fig. 3.2
Neurotrophin receptors, ligands, and functions. Neurotrophins are stored in dense core vesicles (DCV) in hair cells (HC) or supporting cells (SC) at or near a synapse and stimulate both survival and growth in innervating neurons (a). The ear expresses only two neurotrophins (BDNF, NT-3) that signal through their specific receptors (Ntrk2, Ntrk3, Ngfr) in a complex fashion (b). Some in vitro data suggest NT-3 signaling through Ntrk3 (dotted arrows). On dimerized ligand binding, Ntrk receptors activate a complex intracellular cascade via two pathways (Shc pathway, Plcɣ pathway) to support cells by antagonizing the pro-apoptotic function of pro-BDNF, induce growth of cells and processes including some guidance, and play a role in synaptic plasticity. Pro-BDNF can bind to Ngfr to induce apoptosis via ɣ-secretase mediated cleaving of the intracellular death domain (c). Ngfr can also interact with tyrosine kinase receptors (Nrtk’s) to support cell survival through interaction with mature BDNF (c). (Adopted from Panja & Bramham, 2014)
Much like removal of the cochlea has demonstrated the dependency of cochlear nuclei neurons on afferents (Levi-Montalcini, 1949; Harris & Rubel, 2006), transplantation experiments (Ard et al., 1985; Zhou & Van de Water, 1987) and selective deletion of cochlear nucleus neurons (Maricich et al., 2009) indicate that cochlear nuclei support sensory neurons in addition to their support from their peripheral targets. These data suggest that inner ear ganglion neurons are not exclusively dependent on the periphery (i.e., sensory epithelia support sensory neurons which support central neurons) but participate in a more complex interaction involving possible feedback loops whereby both central neurons and sensory epithelia provide support, in turn supported by neurons. Although it is clear that many cochlear nucleus neurons depend on innervation for their viability during a critical phase (Harris & Rubel, 2006), recent data suggest that some cochlear hair cells may also depend on innervation for their long-term maintenance (Kersigo & Fritzsch, 2015). Unfortunately, although retrograde signaling from the target cells across synapses to presynaptic neurons is the most studied signal in the CNS in the context of synaptic plasticity (Panja & Bramham, 2014), such retrograde signals have rarely been considered in the ear (Maricich et al., 2009; Singer et al., 2014).
Nomenclature and abbreviations used throughout this text combined with a summary of effects of genetic deletion are given in Table 3.1.
Table 3.1
Nomenclature and abbreviations used throughout this text combined with a summary of effects of genetic deletion
Gene (mouse) | Aliases | Description | Function revealed by embryonic elimination of the gene |
---|---|---|---|
Atoh1 | Math1 | Atonal homolog 1 | Loss of development of all hair cells |
Bdnf | BDNF | Brain-derived neurotrophic factor | Loss of canal crista innervation, reduction of innervation to apex |
Ngf | NGF | Nerve growth factor | No loss in the ear, loss of autonomic ganglia |
Ngfr | p75NTR | Nerve growth factor receptor | No loss in the ear, reduced motoneuron death |
Ntf3 | NT-3 | Neurotrophin 3 | No loss in the vestibular system, loss of basal turn spiral ganglion neurons |
Ntf4 | NT-4 | Neurotrophin 4 | No loss in the ear, some loss of epibranchial placode derived ganglia |
Ntrk1 | TrkA | Neurotrophic tyrosine kinase, receptor type 1 | No loss in the ear, loss of autonomic ganglia |
Ntrk2 | TrkB | Neurotrophic tyrosine kinase, receptor type 2 | Loss of canal crista innervation, reduction of innervation to apex |
Ntrk3 | TrkC | Neurotrophic tyrosine kinase, receptor type 3 | No loss in the vestibular system, loss of basal turn spiral ganglion neurons |
Plcg1 | PLC–γ | Phospholipase C, gamma 1 | Reduced and misguided innervation of the vestibular system |
Pou4f3 | Brn3c | POU domain, class 4, Transcription factor 3 | Delayed loss of all differentiated hair cells |
Shc1 | SHC | Src homology 2 domain transforming protein 1 | Reduced and misguided innervation of the vestibular system |
Sox2 | Lcc | SRY (sex determining region Y)-box 2 | Loss of all sensory epithelia development of the ear |
3.2 Evolution of Neurotrophins and Their Receptors
As indicated in the introduction, neurotrophins and their receptors are essential for the maintenance of inner ear innervation. Neurotrophins belong to a family of excreted proteins (Hallbook, 1999) that bind as ligands to a set of tyrosine kinase receptors (Hallbook et al., 2006) and the Ngfr (p75NTR) receptor (Bothwell, 2006). The tyrosine kinase receptors mediate their intracellular effects through a phosphorylation cascade (Fig. 3.2) that is integral to promote cell survival and synaptic plasticity (Sciarretta et al., 2010; Bramham & Panja, 2014). Although mammalian nerve growth factor (Ngf) was the first ligand to be isolated and characterized, the later discovered brain-derived nerve growth factor (Bdnf) was subsequently found to be closer to the ancestral neurotrophic ligand out of which the others apparently evolved through gene duplication and mutational diversification (Hallbook, 1999). Bdnf doubled again and these duplications evolved into neurotrophin 3 (Ntf3) and Ngf respectively. A later, additional duplication of Bdnf generated neurotrophin 4 (Ntf4). Bony fishes, with their additional duplication of the whole genome, have several additional neurotrophins. Most interestingly, the expression of bdnf in lampreys is in hair cells of the inner ear (Hallbook et al., 1998), much like in other vertebrates (Pirvola et al., 1992; Farinas et al., 2001), suggesting a historic involvement of this neurotrophin with vertebrate inner ear innervation.
The receptor for BDNF, neurotrophin tyrosine kinase 2 (Ntrk2 or TrkB), appears to be close to the ancestral receptor. The neurotrophin tyrosine kinase 1 receptor (Ntrk1 or TrkA) that binds Ngf and the neurotrophin tyrosine kinase 3 receptor (Ntrk3 or TrkC) that binds NT-3 formed through duplications (Hallbook et al., 2006). In contrast to the ligands, the receptors duplicated only twice and BDNF and NT-4 both signal through the Ntrk2 receptor. In addition, both Ntrk2 and Ntrk3 receptors can produce, by alternative splicing of unique exons, a number of different receptor genes isoforms, including some without an intracellular tyrosine kinase domain used for the canonical signaling. While experimental work has verified that Ntrk2 has indeed only two docking sites on amino acid 515 and 816 (Fig. 3.2) that provide for the intracellular cascade leading to neuronal survival and fiber growth (Sciarretta et al., 2010), docking on amino acid 478 plays additional roles in Ntrk2 signaling (Miyamoto et al., 2006). Likewise, in addition to its pro-survival function, Ntrk3 has additional effects on nerve fiber growth via activation by one of its truncated receptor isoforms (Esteban et al., 2006).
The Ngfr (p75NTR) receptor has a different structure and function compared to the Ntrk receptors (Bothwell, 2006). Although all neurotrophins can signal through this receptor, it is the unprocessed, pro-form of BDNF that appears to be the preferred ligand of Ngfr, collaborating with the co-receptor sortilin for binding (Dekkers et al., 2013). In many systems, the Ngfr receptor, activated by pro-BDNF, acts in a pro-apoptotic way to stimulate cell death and appears to be responsible for some motoneuron death (Taylor et al., 2012). In contrast, mature BDNF binds with higher affinity to Ntrk2 and is clearly anti-apoptotic to rescue neurons as further explained in Sect. 3.3 (Fig. 3.2).
In summary, Bdnf and its Ntrk2 receptor are found in all vertebrates associated with hair cell/sensory neurons and may be close to the ligand–receptor pairing that evolved in early vertebrates, when the single neurosensory cell diversified into a sensory neuron/hair cell pair (Lindholm & Saarma, 2010; Pan et al., 2012b). In contrast, Ntf3 is associated with supporting cells and some hair cells only in the developing mammalian ear whereas its receptor Ntrk3 is already found in the ear-of nonmammals (Pirvola et al., 1997). Neither Ntf4, Ngf, nor the Ngf receptor, Ntrk1, is found in measurable levels in the embryonic ear (Pirvola et al., 1992) and seem to have no role compared to the effects of Bdnf/Ntf3 or Ntrk2/Ntrk3 With this conceptual framework of the role of trophic factors in the support of ear innervation in mind (Figs. 3.1 and 3.2), Sect. 3.3 explores how neurons are supported by and support their central and peripheral targets.
3.3 Embryonic Dependency of Spiral Ganglion Cells on the Cochlea
The vestibular/auditory system of chicken has a natural cell death phase with a loss of about 25 % of inner ear neurons between embryonic days 8 and 14 (E8–14) (Ard & Morest, 1984), about 6 days after the first afferent fibers enter the brain (Fritzsch et al., 1993). This loss partially overlaps with the phase during which central auditory and vestibular nuclei are particularly sensitive to loss of innervation in the chicken (Peusner & Morest, 1977; Rubel & Fritzsch, 2002). This sensitivity on innervation of auditory nuclei seems to continue after hatching (Born & Rubel, 1985). In the rat, degenerating spiral ganglion neurons are most frequent between E18 and E19 (Nikolic et al., 2000), resulting in a loss of approximately 20 % of neurons (Stenqvist et al., 2005). A possible additional loss of 22 % of spiral ganglion neurons (Rueda et al., 1987) occurs in early postnatal mammals when central auditory neurons are particularly vulnerable to loss of innervation (Harris & Rubel, 2006). Experimental data in mice and chicken show that inner ear neurons depend on support from both the hindbrain and the ear, with a combination of both tissues providing the best support (Ard et al., 1985; Zhou & Van de Water, 1987). These developmental data suggest a natural delay of several days between terminal mitosis of sensory neurons and hair cells (Ruben, 1967) and naturally occurring cell death in sensory neurons. Hair cells seem to develop without cell death (Nikolic et al., 2000) even in the absence of all afferents (Ma et al., 2000). Overall, these data fit the neurotrophic theory well, indicating that one population remains constant (hair cells) whereas the projecting ganglion neurons numerically adjusted by about 50 % cell death, much like in many other developing systems (Oppenheim, 1991).
In contrast to these descriptive developmental data, induced neuronal cell death caused by loss of neurotrophins is happening much earlier (Farinas et al., 2001), indicating a limited temporal overlap of natural and experimentally induced cell death. Despite this temporal discrepancy, targeted deletion of neurotrophins or their receptors show that neurotrophic factors, thought to be released primarily from the developing sensory epithelia, are essential for spiral and vestibular ganglion cell survival (Ernfors et al., 1995; Yang et al., 2011; Kersigo & Fritzsch, 2015). Additional support for such a prominent dependency role of sensory neurons on the peripheral target comes from data showing a severe reduction of spiral ganglion neurons in the absence of organ of Corti development (Fritzsch et al., 2005c; Pan et al., 2011). Interestingly enough, the effects caused by the removal of hair cells of the organ of Corti are less profound in terms of embryonic loss of sensory neurons compared to loss of neurotrophins. This suggests that during embryonic development the neurotrophins are all that is needed for neuronal maintenance of cochlear innervation and beyond that, there is no additional support provided by the hair cells or supporting cells. Because all of these effects happen at a time when afferents are growing to the undifferentiated hair cells, there is no possible involvement of synaptic transmission via glutamatergic receptors. In summary, cell death caused by loss of neurotrophins occurs much earlier compared to the peak of natural cell death. In the ear, it is therefore questionable whether such induced cell death is comparable to naturally occurring cell death, which in mammals occurs up to a week later (Rueda et al., 1987; Farinas et al., 2001).
3.4 Embryonic Dependency of Cochlear Nucleus Neurons on Innervation
Whereas neurotrophic dependency of sensory neurons in the developing ear is 100 %, the dependency of cochlear and vestibular nucleus neurons shows dramatic variation in magnitude, onset, and progression after peripheral lesion (Peusner & Morest, 1977; Born & Rubel, 1985; Harris & Rubel, 2006). Even within a given species such as chicken, the magnitude of effects caused by lesions can vary between nearly 50 % in one nucleus to almost nothing in another. In mammals, the magnitude of cochlear nucleus loss depends on the species and the time of afferent lesion (Harris & Rubel, 2006). In addition, the onset of these effects coincides with the beginning of synaptogenesis and may be influenced by synapse formation. However, in some nuclei cell death starts before synaptogenesis (Peusner & Morest, 1977; Petralia et al., 1991; Rubel & Fritzsch, 2002), suggesting different dependence of certain neurons at different times. In fact, cell death shows a critical phase even in individual neurons after ear ablation (Elliott et al., 2015b).
Obviously, 60 years after the effects of loss of innervation on vestibular and cochlear nuclei were first described in the developing chicken (Levi-Montalcini, 1949), our detailed description has improved but our understanding of the variability within and between different nuclei of different species at different time points is still limited. Even within a given nucleus there is profound variation in the responses of individual neurons to the loss of afferents, ruling out simple explanations for dependency effects. New models are needed to clarify these effects at a cellular and a molecular level. Beyond statistical effects across a large cohort of possibly molecularly different neurons reflecting different stages of development, such models should provide correlations of cell death and dendritic growth matching inner ear afferents with cellular gene expression profiles. Such correlative studies could be conducted in aquatic vertebrates as they have a pair of large, bilaterally symmetrical Mauthner cells (neurons that mediate the escape response or c-start) that respond with cell death and dendritic changes to loss of afferents at various times in development (Fritzsch, 1990; Elliott et al., 2015a, b).
3.5 Embryonic Dependency of Hair Cells on Innervation
Obviously, if neurons critically depend on neurotrophins released from hair cells and surrounding supporting cells (Fig. 3.1), one would expect that the initial development of hair cells should not depend on innervation and that hair cells drive, through neurotrophins, the matching of afferents as predicted by the neurotrophic theory (Oppenheim, 1991). Indeed, consistent with this prediction, numerous in vitro and in vivo studies have shown that during embryonic and some postnatal development, hair cells are independent of any innervation (Fritzsch et al., 1997c; Rusch et al., 1998; Ma et al., 2000). However, in other sensory systems such as the taste buds in mammals (Fritzsch et al., 1997b), the electroreceptive organs in fishes (Fritzsch et al., 1990), and the lateral line hair cells in salamanders (Jones & Singer, 1969), there is a variable dependency of the sensory cells on innervation with a fickle time constant. It cannot be ruled out that hair cells of the inner ear might depend on afferent and possibly efferent innervation in the long term as suggested in the very similar lateral line neuromast studies (Jones & Singer, 1969). Unfortunately, such long-term viability tests have not yet been conducted (Rusch et al., 1998), with the possible exception of primary neuropathy in which the etiology is mostly unknown and the loss of neurons may differ between ears (Buchman et al., 2006, 2011). As systemic deletion of neurotrophins or their Ntrk receptors is lethal, cell-type specific conditional deletion of either both Bdnf and Ntf3 or both their receptors, Ntrk2 and Ntrk3, is needed to generate viable mice without any sensory neurons to test the possible dependence of hair cells on innervation. Consistent with these suggestions are recent data on limited long-term hair cell viability after conditional deletion of both neurotrophins (Kersigo & Fritzsch, 2015).
3.6 Late Embryonic and Early Postnatal Development
The postnatal decline of the expression level of neurotrophins (Pirvola et al., 1992), with the exception of Ntf3 in the inner hair cells (IHCs) (Pirvola et al., 1994), suggests that the major function of these ligands is during embryonic development. Overall, the downregulation of neurotrophins relates closely to what has been referred to as the “critical phase” of postnatal development of cochlear nuclei (Harris & Rubel, 2006). Like cochlear nucleus neurons, it is possible that sensory neurons are becoming progressively less dependent on neurotrophin support as they age. Beyond the diminishing role of neurotrophins, there are other effects related to specific differentiation aspects of neurotrophins that are mostly covered in other chapters (see Chaps. 7 by Green et al. and 4 by Davis and Crozier). In Sect. 4.4.1 we outline only two major points of late embryonic and early neonatal function, allegedly associated with neurotrophins: fiber sorting and fiber branching as revealed by complete or targeted ligand deletion or misexpression.
3.6.1 Sorting Two Types of Afferents to Two Types of Hair Cells
All sensory epithelia of amniotes consist of two distinct hair cell types (Lewis et al., 1985). In the vestibular system these are type I and type II hair cells, distinguished by the presence or absence of calyx innervation (Rusch et al., 1998). These two types of hair cells can differentiate without any innervation (Ma et al., 2000). In contrast, the hair cell innervation by a calyx may depend on the level and distribution of neurotrophins, in particular BDNF, the main factor for ganglion neuron survival in the vestibular system (Ernfors et al., 1995). Indeed, calyx formation is disrupted in mutants missing one of the two intracellular pathways downstream of the Ntrk2 receptor for BDNF (Sciarretta et al., 2010). Likewise, the few remaining fibers in Bdnf null mice (Bdnf −/−) show only occasional and partial formation of calyces indicating that the presence of BDNF, and to a lesser degree of NT-3, drives calyx formation. The process by which this is achieved is unclear, but it may relate to the well-known function of BDNF in long-term potentiation (LTP) plasticity in the developing and adult nervous system (Bramham & Panja, 2014).
The mammalian organ of Corti also has two distinct types of hair cells. In contrast to the random distribution of vestibular hair cell types, cochlear hair cell types are very orderly and differentially distributed into one row of inner hair cells (IHCs) and three rows of outer hair cells (OHCs). Each cochlear hair cell type is innervated by a different set of spiral ganglion neurons. Numerous theories have been proposed as to how the type I spiral ganglion afferents organize the innervation of 10–30 fibers on a single IHC and the growth of type II afferents to OHCs, innervating 20 or more OHCs by a single fiber (Simmons et al., 2011; Bulankina & Moser, 2012), including a possible function of neurotrophins and their receptors. Early work suggested a simple correlation: NT-3 and Ntrk2 regulate innervation to IHCs whereas BDNF and Ntrk2 regulate the innervation to OHCs (Ernfors et al., 1995; Schimmang et al., 1995). It remained unclear how such simple correlations can be reconciled with the overlapping expression of Ntrk2 and Ntrk3 in spiral ganglion neurons and of Bdnf in all hair cells (Pirvola et al., 1992; Ylikoski et al., 1993; Farinas et al., 2001). In contrast to these simple correlations of one neurotrophin/neurotrophin receptor with a given pairing of spiral ganglion cell/hair cell type, other work showed a sophisticated change in expression (Fig. 3.3) during the growth of afferents (Fritzsch et al., 1995, 1997a). Follow-up work using mouse models with replacement of either Ntf3 with Bdnf [Ntf3 tgBdnf (Coppola et al., 2001; Tessarollo et al., 2004)] or Bdnf with Ntf3 [Bdnf tgNtf3 (Agerman et al., 2003)] revealed functional equivalence of BDNF and NT-3 during most inner ear innervation development. However, more recent work suggested that the differential density of IHC and OHC innervation may be related to quantitative expression differences of neurotrophins (Yang et al., 2011). It is possible that single IHC innervation by 10–30 type I spiral ganglion neurons may depend on the late embryonic coexpression of Ntf3 and Bdnf in IHCs. In contrast, type II fibers contact many OHCs, possibly to accumulate enough BDNF for viability. Clearly, either Bdnf (Farinas et al., 2001; Tessarollo et al., 2004) or Ntf3 expression (Agerman et al., 2003) in OHCs can provide enough support to maintain type II fibers. However, neurotrophins are unlikely candidates to explain why these fibers grow to the outer compartment of the organ of Corti in the first place. Obviously, the high level of expression of both neurotrophins in and around IHCs (Fig. 3.3) would argue that they should remain in the inner compartment of the cochlea.
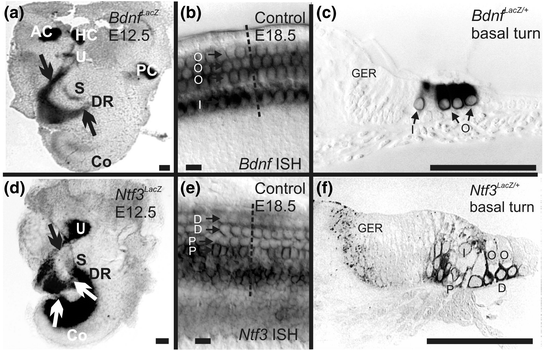
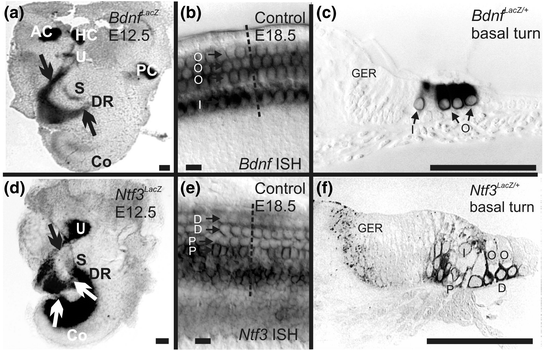
Fig. 3.3
Neurotrophin and neurotrophin receptor expression revealed by ß-galactosidase staining in LacZ-knockin mice and in situ hybridization. Bdnf is expressed early on in the three canal cristae (anterior crista, AC; horizontal crista, HC; posterior crista, PC); the early differentiating hair cells of the utricle (U) and saccule (S), and the apex of the cochlea (Co); and in delaminating neurons (arrows in a). Later Bdnf is primarily expressed in hair cells, with limited expression in supporting cells (b, c). In contrast, Ntf3 is not expressed in early embryonic canal cristae but shows strong expression in the utricle, saccule and the basal turn of the cochlea as well as in delaminating neurons (arrows in d). In older mouse embryos, Ntf3 is primarily expressed in supporting cells of the cochlea (e, f) and the IHCs (I) but not in the OHCs (O). D, Deiters’ cells; DR, ductus reuniens; GER, greater epithelial ridge; P, pillar cell (Compiled after Farinas et al., 2001). Bar indicates 100 µm (a, c, d, f) and 20 µm (b, e)
Nevertheless, the sorting of afferent fibers to various hair cell types has superficial resemblance to the basic assumption of the neurotrophic theory to achieve numerical matching of source and target (Oppenheim, 1991). However, closer examination reveals that simple numerical matching of fibers to target(s) is difficult to apply in the ear. In contrast to other developing systems, such as skeletal muscle fibers (Oppenheim, 1989), the ear shows variable ratios of afferents to hair cells, all seemingly related to the variable distribution of just two neurotrophins expressed during the time of fiber sorting in late embryos and early neonates:
1.
In the vestibular system, there can be a 1:1 ratio of afferents to type I hair cells. Whereas calyces are found throughout the vestibular epithelia, calyces without afferent branches are associated with the striola region in the utricle and saccule (Desai et al., 2005b), which expresses Ntf3 and Bdnf early in development (Farinas et al., 2001). It is conceivable that the coexpression of Ntf3 and Bdnf allows this unusual 1:1 ratio to develop whereas afferents outside the coexpression zone of Bdnf and Ntf3 form additional branches to many more hair cells [mixed afferents (Desai et al., 2005b)]. The formation of some calyces in Bdnf null mutants (Bdnf −/−) as well as in Ntrk2 point mutants [Ntrk2 PLC/PLC (Sciarretta et al., 2010)] is consistent with this hypothesis.
2.
In the vestibular type II hair cells and the OHCs of the organ of Corti, a single fiber can converge on multiple hair cells (Fritzsch, 2003; Desai et al., 2005a; Nayagam et al., 2011). This aspect is particularly difficult to reconcile with the neurotrophic theory as it indicates a highly variable numerical match or no obvious match at all.
3.
In the IHCs of the organ of Corti, 10–30 or more type I afferent fibers converge onto a single IHC (Nayagam et al., 2011).
Clearly, the highly variable innervation density of different inner ear hair cells conflicts with a simple quantitative afferent-to-target ratio sorting in the ear, as specified in the original formulation of the neurotrophic theory. Obviously, if multiple afferents can be supported by a single hair cell, there is no easy explanation why only a single afferent is supported by other hair cells and multiple hair cells are needed to support yet another set of afferents. However, the complex qualitative and quantitative expression of the two neurotrophic factors and their receptors could accommodate for such variable numerical ratios between fibers and hair cells. Further assessments combining conditional deletion with misexpression of neurotrophins (Agerman et al., 2003; Tessarollo et al., 2004) are needed to resolve these interactions beyond the limited available data supporting such a notion (Yang et al., 2011). Most recent data demonstrate that induced overshooting of afferents beyond areas of hair cells is not corrected through elimination of these fibers (Mao et al., 2014), indicating limits to redirecting misguided fibers in the ear, clearly not in agreement with the neurotrophic theory (Oppenheim, 1991). In summary, while overall the ear appears to be an ideal system to establish the basic assumptions of the neurotrophic theory, the details uncovered thus far do not support that “programmed” cell death or pruning of fibers to eliminate aberrant projections is at all easily applicable in the ear.
3.6.2 Promoting Branching and Targeting in the Cochlear Nuclei
Both spiral ganglion fiber types terminate adjacent in the cochlear nucleus despite their very different peripheral distribution (Nayagam et al., 2011). Afferent growth into the cochlear nuclei occurs early and follows a simple temporal order: the basal spiral ganglion neurons become postmitotic first and reach the cochlear nucleus first, branching to reach all subdivisions (Fritzsch, 2003; Matei et al., 2005; Fritzsch et al., 2014). Consistent with other developing hindbrain sensory systems (Fritzsch et al., 1997b, 2005a), initial projection to cochlear nuclei develop prior to hair cell differentiation and can progress in their absence (Xiang et al., 2003). Although some immunocytochemical data suggest the presence of both neurotrophins at different times in the cochlear and vestibular nuclei, in situ hybridization shows that only Ntf3 is expressed in the embryonic cochlear nuclei. Obviously, this neurotrophin could provide additional support to spiral ganglion afferents consistent with data indicating that the cochlear nuclei provide some neurotrophic support (Maricich et al., 2009). It is possible that the presence of neurotrophins in the target nuclei can affect the branching proportional to the overall vitality of neurons [better supported neurons branch more profusely (Elliott et al., 2015b)] as compared to more specific branching effects. In contrast, embryonic vestibular nucleus neurons seem to express very little if any neurotrophins, suggesting that expression of neurotrophins in the cochlear nucleus is unique to that nucleus and is possibly related to the unique molecular development of these nuclei, distinct from vestibular nuclei (Fritzsch et al., 2006; Duncan & Fritzsch, 2013). Deletion of neurotrophins only in the ear or only in the cochlear nucleus is needed to dissect the molecular basis of the sorting processes and to expand the role of neurotrophins in conjunction with spontaneous activity (Marrs & Spirou, 2012).
3.7 Embryonic Expression of Neurotrophins and Their Receptors
While embryonic viability of all inner ear neurons hinges on only two neurotrophins and their receptors (Ernfors et al., 1995; Fritzsch et al., 1997c), losing only one or the other neurotrophin or its receptor leads to complicated patterns of incomplete loss of inner ear neurons and complicated patterns of residual innervation (Fig. 3.4). Understanding the differential effects of spiral ganglion and vestibular neuronal loss is seemingly directly related to the differential expression of the two neurotrophins (Fig. 3.3) that support all inner ear sensory neurons during embryonic development. Thus, whereas loss of Bdnf and Ntf3 shows distinct effects in the vestibular system, their loss in the cochlea may be largely quantitative, reflecting their differential expression levels during development (Yang et al., 2011) rather than a unique function. Understanding the data around the common and unique function of neurotrophins requires some background information on the structure and function of these ligands.