CHAPTER 132 Neural Plasticity in Otology
In the following sections, we carefully define what we mean by plastic change in otology. We discuss different types of plasticity and introduce the important distinction between developmental plasticity and adult plasticity. We then consider in detail experimental studies that serve to illustrate key aspects of plasticity in the auditory system. This is followed by a review of some important underlying mechanisms of plasticity. We consider these mechanisms at a systems physiology level, and at more basic cellular and subcellular levels. The review is far from exhaustive, and can be supplemented by other key studies1–8 and the more comprehensive textbooks listed under Suggested Readings. In the final section, we discuss what this new knowledge about sensory system plasticity means to the otologist or hearing health care professional.
Definition of Plasticity
A broad definition of plasticity, as it pertains to otology, is the inherent ability of the auditory (or vestibular) system to modify or reorganize. The term “plasticity” is used to cover a wide spectrum of phenomena, and it can mean different things to different people. Consider the variety of experimental methods that have been used to reveal plastic change in the auditory system. Various anatomic studies have established that plasticity relates to physical change in the brain. Some of the earliest observations made, including the pioneering work of Levi-Montalcini,9 were of the anatomic changes to auditory pathways after experimental lesions to the inner ear. In such studies, cell counts, axonal pathway changes, and alterations to neural structure were observed to follow partial or total auditory deafferentation. Further examples of anatomic studies of plasticity in the auditory system are given in a subsequent section.
The research literature also contains many physiologic studies in which short-term or long-term change in central auditory system function has been observed after experimental manipulations to auditory input. In this chapter, many of these studies are presented in detail. The methods for the evaluation of plasticity of auditory function include various electrophysiologic studies in animal models (see later sections) and in humans,10,11 and functional neuroimaging methodologies. The latter include positron emission tomography, functional magnetic resonance imaging, and magnetoencephalographic studies.12,13
Time Course of Plastic Change
The term “plasticity” is currently used in auditory neuroscience to describe phenomena that differ considerably in their time courses. Plasticity is used to describe changes to the auditory system occurring very rapidly, over a span measured in minutes, and much longer term changes over weeks, months, and years. As an example of acute plasticity, auditory neuron receptive fields (excitatory and inhibitory frequency tuning curves) have been observed to change within 10 minutes after induction of cochlear lesions or partial deafferentation.14,15 It is reasonable to suppose that short-term plastic change reflects small-scale neural alterations, with subtle synaptic modifications to existing neural networks. With such acute observations, initial stages of plastic change may have occurred, but it is unclear whether these changes would be consolidated in the longer term (see the section on basic mechanisms of plastic change).
More extensive auditory system reorganization seems to occur over a longer time course. Numerous studies show a modification of central tonotopic mapping as a result of cochlear lesions or partial deafferentation.16–21 In these studies, the time course of plastic change apparently is completed over many weeks. In such cases, underlying synaptic strengthening seems to be consolidated. Sometimes very extensive reorganization is observed, which suggests that long-term processes, such as new axonal growth and synaptogenesis, have occurred. To use a computer analogy, short-term plasticity might be considered to be software changes, whereas long-term changes might also include hardwiring modifications.
Age-Related Plasticity
Perhaps the most important factor to consider when reviewing studies relating to plasticity is the age, or rather the developmental state, of the subject. As shown in subsequent examples, reorganization of tonotopic maps after cochlear lesions is significantly different when experimental manipulation is done in the neonate compared with the adult animal. There are numerous other examples of age-related plasticity in the auditory system. Perhaps the most familiar to the otologist is the performance of a congenitally deaf child provided with a cochlear implant at an early age compared with a deaf child implanted at a much later age.22 Studies such as this and other clinically related aspects of auditory system plasticity are explored in the final section of this chapter, where we consider what all this new knowledge about plasticity means to the practitioner.
Experiments Showing Plastic Change in Otology
Historical Background
Two pioneering studies drew attention to the notion that early sensory input has a crucial role in central brain development. These two experiments paved the way for many modern studies of sensory brain plasticity. The seminal study was in the developing chick auditory system by Levi-Montalcini,9 who documented the anatomic changes to central auditory pathways after the otocyst of the chick embryo was removed or damaged. In later, seemingly unrelated studies, Wiesel and Hubel23,24 showed that visual cortical wiring responsible for ocular dominance columns is disrupted in cats if, during an early postnatal period, the animals have had visual input from one eye only (i.e., after neonatal monocular deprivation).
These seminal experiments were followed by many others in visual, somatosensory, vestibular, and auditory systems. In otologic research, numerous studies revealed the considerable anatomic changes that result from neonatal ablation of one cochlea. These changes include loss, or pathologic change, to neurons in brainstem and midbrain,25–27 and, importantly, the formation of novel innervation patterns, particularly from the cochlear nucleus on the normal side to more central nuclei.28,29
Other relevant studies were made in other sensory systems. The visual experiments by Wiesel and Hubel23,24 have already been mentioned. In addition, work in the somatosensory system pointed the way to analogous experiments in the auditory system. The reorganization of somatosensory maps in cortex after damage or partial deafferentation of the sensory inputs was initially shown after whisker removal in young rodents,30 and after peripheral nerve damage or digit removal in developing and adult animals.31–33 These experiments were significant because they noted reorganization of the cortical somatotopic maps, which can be considered, as Merzenich and associates32 would say, the “mainline functional organization” of this sensory system. These studies also showed that deafferented cortical areas (i.e., no longer receiving input from the periphery) become connected to areas corresponding to the border of the peripheral lesion. In terms of cortical space, these border areas become overrepresented. As discussed in the following sections, there are considerable similarities between the somatosensory data and data from analogous experiments in the auditory system.
Reorganization of Central Tonotopic Maps after Cochlear Lesions
The main experimental studies I have chosen to illustrate “plastic change in otology” are those in which central tonotopic map reorganization occurs after lesions are made to the cochlea. These experiments show how peripheral activity patterns can influence the establishment and maintenance of central frequency maps. Analogous to the central somatotopic projections mentioned previously, tonotopic maps can be considered the mainline organizational feature of the auditory system. In the auditory system, the topographic order of afferent neurons is well maintained from the sensory epithelium of the cochlea up to the cortex.34,35 Strictly speaking, we should refer to this projection system as “cochleotopic,” analogous to the similarly organized retinotopic visual system and the somatotopic pathways of the somatosensory system. The interchangeability of “cochleotopic” and “tonotopic” is possible because of the place coding of sound frequency along the cochlear length. Some caution is advised, however, on the interchangeability of these terms when considering systems having pathologic cochleas; under such conditions, considerable distortion of the normal place coding of sound frequency is possible.
To set the scene for the experimental studies that follow, let us consider the normal cochleotopic (tonotopic) mapping at the level of the auditory midbrain and cortex. Figure 132-1 shows a tonotopic map in the primary auditory cortex of a cat, as determined by recording from cortical neurons at several sites.18 Each data point indicates the microelectrode recording site, and the sound frequency to which neurons respond best is indicated in kilohertz. The lower panels of Figure 132-1 indicate how the response of (three) individual neurons is analyzed. Each raster shows the response (spike count) to stimuli of different frequency and intensity combinations. The isofrequency contours that are drawn through the cortical map are plotted at octave intervals. Similar microelectrode recording techniques were used to determine tonotopic maps in the inferior colliculus of the midbrain. Such a map from a normal chinchilla is shown in Figure 132-2A.16
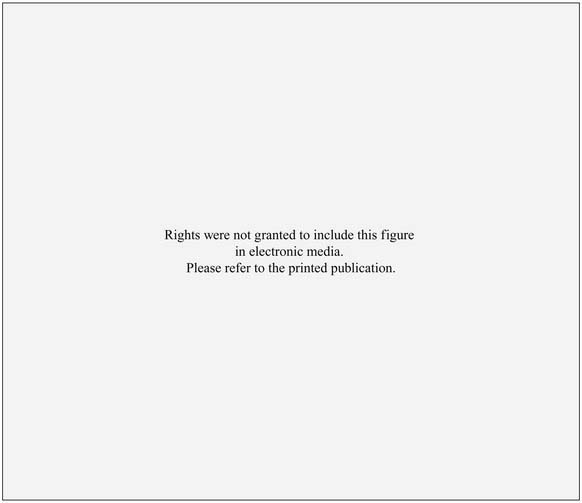
Figure 132-2. Tonotopic maps in the inferior colliculus (central nucleus) of a chinchilla. All maps were derived from single neuron recordings in electrode tracks along the dorsoventral axis of the inferior colliculus as shown. Neuron characteristic frequency was derived from receptive field plots similar to those shown in the cat experiments of Figure 132-1. The upper graphs plot neuron characteristic frequency as a function of electrode excursion. The lower diagrams represent the tonotopic maps; isofrequency contours are indicated at octave intervals. A, Results from a normal adult subject. B, In this subject, a basal cochlear lesion was made within days of birth, and the inferior colliculus tonotopic map was derived 4 months later. In the map, the shaded area shows the ventral region of the inferior colliculus in which all neurons had a common characteristic frequency. C, In this subject, the cochlear lesion was made in the adult animal and was mapped 3 weeks later.
(Adapted from Harrison RV, Ibrahim D, Mount RJ. Plasticity of tonotopic maps in auditory midbrain following partial cochlear damage in the developing chinchilla. Exp Brain Res. 1998;123:449-460.)
Tonotopic Map Reorganization in a Developmental Model
Figure 132-3 shows two examples of cortical tonotopic maps in cats treated shortly after birth with the ototoxic aminoglycoside amikacin.18 This treatment resulted in basal cochlear hair cell lesions. Amikacin was given systemically, and cochlear lesions were bilaterally symmetric. Histologic evaluation in subject A showed that the basal region of the cochlea was totally damaged (inner and outer hair cell loss), but in more apical areas, above the 6- to 8-kHz region, a normal sensory epithelium was present. This is consistent with the auditory brainstem response (ABR)–derived audiogram showing normal tone pip evoked ABR thresholds up to the high-frequency cutoff slope. The cortical tonotopic map for this subject is characterized by a normal representation of low frequencies, but the cortical region that has been deprived of normal input by the partial cochlear deafferentation now contains neurons that are all tuned to 6 to 8 kHz (shown as the shaded area). The boundary region of the cochlear lesion is abnormally overrepresented in terms of cortical space.
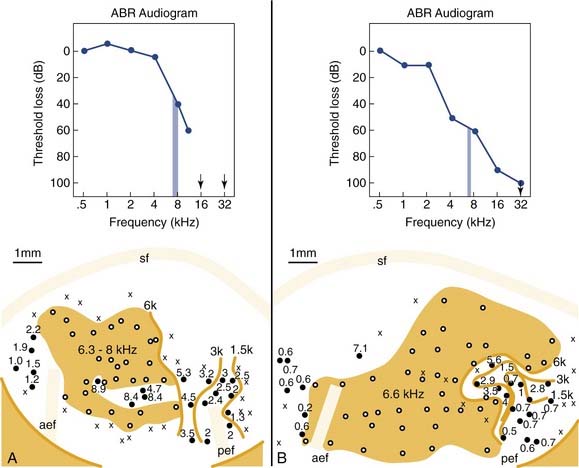
Figure 132-3. Cortical tonotopic maps that developed in two cats with neonatal basal cochlear lesions. The kittens were treated with the ototoxic aminoglycoside amikacin to produce basal lesions to the cochlea. The effects of such lesions are reflected in the auditory brainstem response (ABR)–derived audiograms (upper panels). A, In this subject, the cochlear lesion was confined to the basal region. B, In this subject, hair cell damage was maximal at the cochlear base, but scattered hair cell loss extended up to apical regions. Tonotopic maps are plotted as for Figure 132-1. Isofrequency contours are at octave intervals. Shading indicates regions in which all neurons have similar tuning properties.
(Adapted from Harrison RV, Nagasawa A, Smith DW, et al. Reorganization of auditory cortex after neonatal high frequency cochlear hearing loss. Hear Res. 1991;54:11-19.)
In subject B (see Fig. 132-3B), the results are more revealing. In this kitten, the cochlear lesion was more extensive, with a severe basal lesion, but also with scattered hair cell loss up to apical regions. This is reflected in the ABR audiogram that gradually slopes down across all frequencies measured. This kitten also developed a cortical frequency map in which there was a very large isofrequency area (shown as a shaded area) where all neurons have common 6.6-kHz frequency tuning. In addition, the low-frequency region of the tonotopic map is severely distorted compared with normal (compare with Fig. 132-1). It seems that a reduced or abnormal stimulus-driven activity pattern from cochlear areas more apical to the main lesion has had an influence on normal map development.
Cortical Frequency Map Reorganization in Adult Subjects
The experimental results shown in Figure 132-3 were from studies in which the cochlear lesions were induced in neonates.18 Qualitatively, similar results are also found at the cortical level if the lesions are made in an adult animal. This was initially reported by Robertson and Irvine,21 who were the first authors to report observations of altered central tonotopic maps resulting from peripheral lesions. Other studies subsequently confirmed these findings in adult animals.19,20,36
Figure 132-4 shows results from a chinchilla. Here a normal tonotopic map (Fig. 132-4A) is compared with a map recorded from an adult animal (Fig. 132-4B) with cochlear lesions induced.19 The anatomic extent of the lesion is shown on the cochleogram, and the corresponding ABR audiogram shows the functional consequences. A typical finding is an overrepresentation of neurons with characteristic frequency of approximately 2.5 to 3.5 kHz (shaded area). In the adult animal, as with the developing subject, neurons in deafferented cortical regions seem to become connected to adjacent noninterrupted ascending input. Similar principles of adult cortical map reorganization are found in somatosensory and visual systems.2,37,38
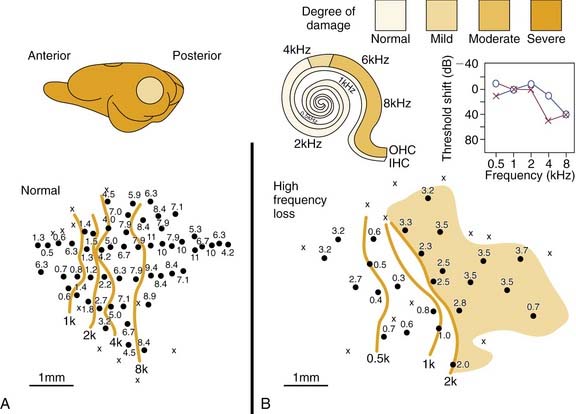
Figure 132-4. Cortical tonotopic maps in a normal adult chinchilla (A) and in a subject 4 weeks after making cochlear lesions by amikacin treatment (B). Methods for cortical maps were derived with methods similar to those described for Figures 132-1 and 132-3. Isofrequency contours are octave spaced. The peripheral deficit in the treated chinchilla (B) is reflected in the cochleogram and in the auditory brainstem response audiogram. The shaded area in the abnormal cortical map indicates the regions in which most neurons had very similar tuning properties. IHC, inner hair cell; OHC, outer hair cell.
(Adapted from Kakigi A, Hirakawa H, Mount RJ, et al. Tonotopic mapping in auditory cortex of the adult chinchilla with amikacin-induced cochlear lesions. Audiology. 2000;39:153-160.)
These modifications to cortical frequency maps seem to be the result of neural rewiring, not an experimental artifact. The excitatory receptive fields of neurons tuned to the common frequency are qualitatively similar in shape. They have similar minimum thresholds and bandwidths, independent of where they are located across cortical space. This is an important observation because it argues against the possibility that the common characteristic frequency is an artifact caused by simply truncating all neuronal tuning curves above a certain frequency (corresponding to the lesion boundary along the cochlear sensory epithelium). This possible “pseudoplasticity” (i.e., the appearance of reorganization when there is really none) has been discussed by Kaltenbach and colleagues.39
Adult versus Developmental Plasticity of Sensory Systems
The importance of early sensory stimulation in driving the development of the mature brain has been emphasized by many investigators starting with Wiesel and Hubel23,24 and reinforced by other experiments in various sensory systems.3,31,40,41 At the level of auditory cortex, there seems to be a pattern of reorganization of tonotopic maps after cochlear lesions induced in the developing neonate similar to that of the adult animal. Intuitively, one might expect considerable differences in the extent of the cortical tonotopic map reorganization in subjects with lesions made in early development compared with lesions made in adulthood. Qualitatively, this seems to be the case,19 but there have been no careful within-species studies to answer this question specifically. If we explore tonotopic map reorganization in subcortical auditory nuclei, however, there are significant differences in map reorganization after lesion experiments in a developmental model compared with the mature subject. At subcortical levels, we see evidence of age-related plasticity.