Fig. 47.1
OCT images of fresh, ex vivo porcine eyes after intra-cameral injection of contrast agents. From top to bottom: Control, triamcinolone acetonide 40 mg/mL, prednisolone acetate 1 %, and lipid-based artificial tears. The agents provide positive contrast within the anterior chamber that has a different pattern depending on the type of agent used. These differences are expected because the agents are comprised of varying particulate or oil-in-water suspensions, with differing mean particle sizes, reflectivities, and diffusivities
While scattering-based contrast agents are readily visible within the highly transparent anterior segment of the eye, the ability to detect these types of agents endoscopically or on the skin is more challenging, as they must be distinguishable against the already high optical scattering of the tissue. Mie theory provides exact solutions for light scattering from spherical particles, shelled spheres, and spheroids [11]. As a general rule, there is a rapid increase in scattering with particle size (scattering cross section, σs ∝ d 6) in the Rayleigh regime (d << λ) and a rapid increase in scattering as the material refractive index is different (higher or lower) than that of the (typically aqueous) medium. At the same time, one must weigh the choice of the material and the particle size against the biocompatibility and the ability for the probes to access their target, respectively, for the needed application.
One of the most useful types of OCT contrast agents, which will be discussed many times throughout this chapter, is plasmonic gold nanoparticles in their various forms (nanospheres, nanoshells, nanorods, etc.). This is because gold is highly unreactive and consequently relatively biocompatible, while at the same time providing a surface plasmon resonance (SPR) effect at the red and near-infrared wavelengths used in OCT [12]. This SPR is evident as either a strong absorption or scattering spectral peak, with a transition from predominantly absorbing (low albedo) to predominantly scattering behavior (high albedo) as the particle size is increased; for spheres, the transition occurs at d ≈ 80 nm [12]. Nanoshells in particular have been highly developed for scattering-based OCT contrast [13–15]. They are comprised of a silica core and gold shell and offer spectral tunability by adjusting the core diameter and shell thickness [3]. Figure 47.2 displays a demonstration of enhanced OCT contrast in the tumors of mice systemically intravenously injected with nanoshells [13]. These results highlight the enhanced permeation and retention (EPR) effect, whereby the permeable vasculature of tumors acts to trap particles, providing selective targeting [16]. Importantly, EPR further enables site-targeted treatment; in this example, the nanoshells were designed to be nearly equally light scattering and absorbing, providing both imaging contrast (via scattering) and photothermal therapy (via absorption).
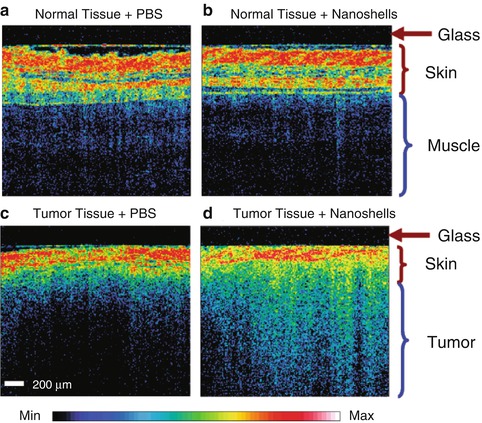
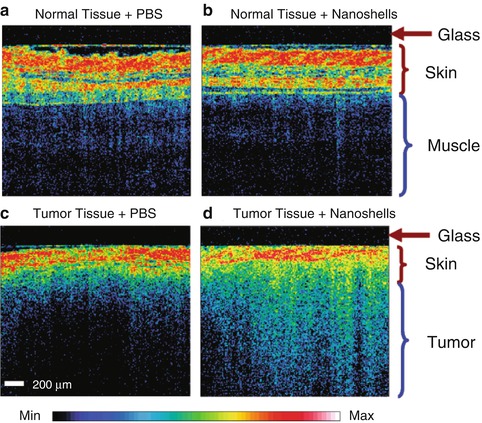
Fig. 47.2
OCT images of tissues from mice with subcutaneous tumors systemically treated with phosphate-buffered saline (PBS) as a control (a, c) and multifunctional nanoshells (b, d). Enhanced retention of nanoshells in the tumor in panel (d) provides better delineation of tumor borders, as well as subsequent tumor-specific photothermal ablation (Reprinted with permission from Ref. [13]. Copyright 2007 American Chemical Society)
It should also be noted that, in cases where the SPR is narrow compared to the bandwidth of the light used in OCT, it may be possible to employ spectroscopic OCT (SOCT) techniques to distinguish the SPR signature, providing enhanced specificity against the tissue scattering background. While this idea has been explored [17], a confounding factor that makes this method challenging is the highly modulated backscattering spectrum typically obtained from Mie scatterers (d ∼ λ) within the tissue. In current practice, SOCT techniques are much more commonly employed to detect absorption-based contrast agents, which is the focus of the following section.
47.3 Absorption-Based Contrast
Light absorption is a very attractive molecular process to exploit for contrast, both because of the potential signal strength and because essentially all molecular species have the capacity to absorb light. The imaging light used in OCT is spectrally broad, and hence, the backscattered spectrum may be utilized to identify the absorption spectrum of endogenous or exogenous species present within the tissue. The group of techniques designed to extract this information are collectively referred to as spectroscopic optical coherence tomography (SOCT) [18].
The different algorithms developed for SOCT diverge in how they deal with the trade-off between spatial and spectral resolution. One approach is to use multiple light sources to collect independent OCT images with different center wavelengths. Relatively straightforward algorithms such as spectral triangulation [19] may then be implemented to extract the depth-resolved backscattered spectrum. A judicious choice of center wavelengths can facilitate the detection of highly peaked spectral features with limited spectral resolution. This approach has largely been used to detect dyes such as indocyanine green (ICG) [19].
An alternate approach is to directly utilize the broad spectral bandwidth of the light source and use the short-time Fourier transform (STFT) to gain spectral resolution at the expense of spatial resolution [20]. This approach has the advantage that it is entirely a post-processing technique; hence, it can be tailored to maximize contrast to a target contrast agent. Likewise, the time-frequency distribution (TFD) need not be limited to the STFT but may optimized as well to maximize the spatial and spectral resolution [21]. Wax and coworkers [22] have recently developed an algorithm that incorporates two STFTs, one with a narrow spectral window and one with a broad spectral window. The two TFDs are multiplied point by point to generate a TFD with both high spatial and spectral resolution.
Exogenous chromophores for SOCT are largely repurposed, commercially available fluorescent dyes. Utilizing these dyes carries with it the advantage of a wealth of biological and chemical research aimed toward targeting particular disease states of tissue, chemical species, or morphologies. Some examples include ICG [23], photodynamic therapy-related dyes [24], and fluorescent microspheres [25]. Dyes typically also have strongly peaked spectra which enable detection via fairly simple methodologies. For instance, a commercial NIR absorbing dye (H.W. Sands, ADS7460) which exhibits a sharp absorption peak at 740 nm was used to produce contrast in an 800 nm OCT system by effectively clipping the shorter wavelengths, resulting in a redshift of scattered light [26].
The major endogenous chromophore is hemoglobin. Detection of hemoglobin absorption with SOCT has been explored as a method to measure blood oxygen saturation [27]. Wax and coworkers [22] recently measured the oxygen saturation along with fluorescein dye injected into the bloodstream in a mouse window chamber model using METRiCS OCT which uses the two TFD methods noted above along with OCT imaging at nontraditional wavelengths. Their imaging bandwidth spans the 455-695 nm range which overlaps strong peaks in the oxy- and deoxyhemoglobin spectrum. Selected results from this work demonstrating the endogenous and exogenous tissue contrast as well as SO2 measurements are shown in Figs. 47.3 and 47.4.
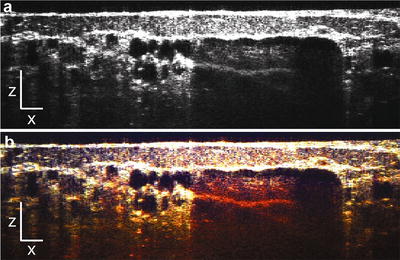
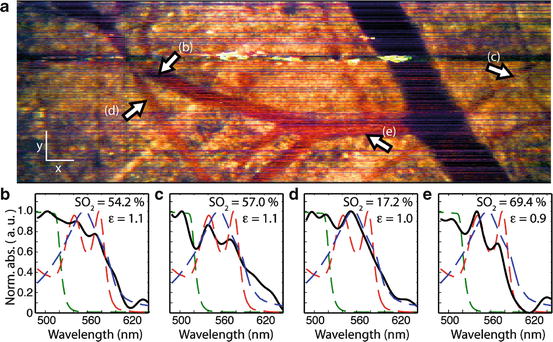
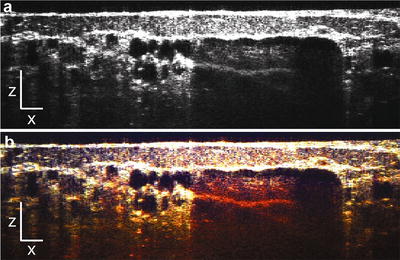
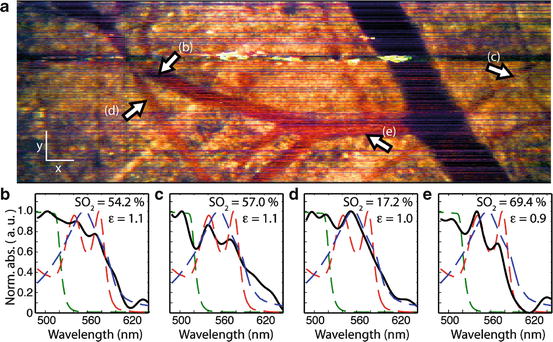
Fig. 47.4
(a) En face METRiCS OCT image with arrows indicating points where the spectra are extracted. White x and y scale bars, 100 μm. (b–e) Spectral profiles from corresponding points in (a). Measured spectral profiles (black) are superposed with the theoretical oxy- (dashed red) and deoxy- (dashed blue) hemoglobin normalized extinction coefficients, and normalized absorption of NaFS (dashed green). Also shown are the SO2 levels and the relative absorption of NaFS with respect to total hemoglobin (ε ≡ NaFS/Hb). All spectra were selected from depths immediately below each corresponding vessel (Reprinted with permission from Ref. [22]. Copyright 2011 Macmillan)
Plasmonic gold nanoparticles have also been widely employed for absorption-based contrast, where the SPR peaks are tuned within the OCT imaging band by varying the size of specific geometrical features of the nanoparticles. For instance, light-absorbing gold nanorods are tuned by varying their aspect ratio (length over width) while maintaining a length typically <100 nm to favor absorption over scattering. Many of the different particle geometries have been explored for contrast in SOCT, including gold nanospheres [28], gold nanorods [28, 29], and gold nanocages [30]. For example, gold nanorods were imaged after injection into excised human breast carcinoma tissue [29]. As mentioned above, light-absorbing gold nanoparticles are, at the same time highly effective for photothermal cancer therapy, where a high power laser is used to irradiate particle-laden tumors [31]. The synergy between imaging and therapy, which allows us to monitor permeation and diffusion of SPR particles into tissues before treatment, aids in particle development for improved delivery and informs the design of more effective treatment protocols.
47.4 Pump-Probe OCT
Pump-probe optical coherence tomography (PPOCT) is fundamentally the fusion of optical coherence tomography with pump-probe absorption spectroscopy. Spatially resolving the pump-probe interaction can provide molecular contrast for absorbing agents in a tissue sample similar to SOCT. The major advantages of PPOCT are that contributions to the OCT light attenuation due to scattering and absorption are easily separated and there is no compromise between spatial and spectral resolution. The disadvantages are that the optical setup is more complicated and typically requires pulsed light sources. Only under special circumstances could PPOCT be accomplished with a swept OCT laser source or superluminescent diode.
A typical PPOCT system has the following features: The probe is the light in the sample arm of the OCT interferometer, i.e., the same light used for OCT imaging serves as the probe light. A separate pump beam co-propagates with the OCT light in the sample arm. The pump is typically amplitude modulated at frequency f 0. Transfer of the modulation onto the backscattered probe (OCT) signal at f 0 is then evidence of absorption of the probe light by some tissue absorber. In time-domain OCT implementations, the PPOCT signal appears as sidebands on the Doppler carrier frequency, f D ± f 0 [32]. In spectral-domain OCT, the PPOCT signal can be extracted from an M-scan by Fourier transformation along the time axis (at each depth) and filtering around f 0. For the process to work, absorption of the pump by the contrast agent must change the absorption/scattering properties at the probe wavelength.
The first experimental realization of PPOCT [33] demonstrated imaging of methylene blue, a dye used for chromoendoscopy [34]. The specific physical mechanism leading to PPOCT signal from methylene blue is well understood and therefore serves as a germane example, where the energy level diagram is illustrated in Fig. 47.5a. The pump light drives a transition from the singlet ground state (S0) to the first excited electronic state (S1). Molecular population in the excited singlet state is transferred to the triplet state via a particularly efficient [35] spontaneous process (S1 → T1, τ1-1). Methylene blue in its triplet state has a resonant transition peaked at 830 nm (T1 → T2). When the pump is on, an 830 nm probe can be absorbed by methylene blue, but when the pump is off, there is no probe absorption. In reality the excited triplet state has a finite lifetime (analogous to fluorescence lifetime) that is a function of the oxygen level in its local environment, but varies from ∼200 ns to over 1 μs. Consequently, the pump and probe need not be incident on the sample at the same time, but may be delayed in time by some fraction of the excited state lifetime. Measurement of this characteristic lifetime may be used to help differentiate among multiple chromophores. An example decay for methylene blue is in Fig. 47.5b. The average decay time (lifetime) from T1 to S0 (τ0−1) was calculated to be 247 ns via τ avg = ∑ S ⋅ t/∑S, where S is the PPOCT signal at delay time t [36]. In addition to the lifetime, the absorption spectrum at the pump or probe may be measured by recording the PPOCT signal as a function of the pump or probe wavelength, respectively.
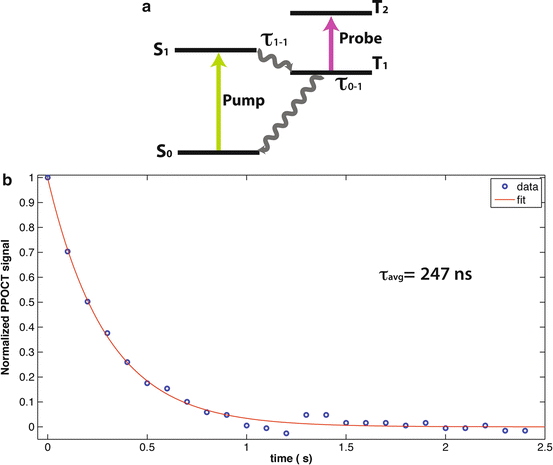
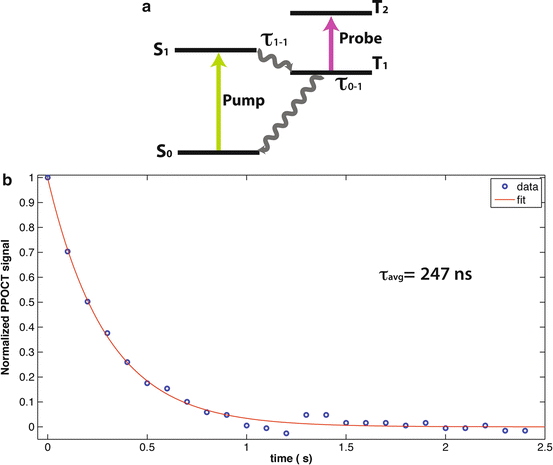
Fig. 47.5
(a) Molecular energy level diagram for the methylene blue PPOCT mechanism. Driven transitions are indicated by straight arrows and spontaneous transitions as zigzag arrows. (b) Measured normalized decay of the PPOCT signal due to methylene blue as a function of delay between the pump and probe pulse. The decay has a characteristic average lifetime of 247 ns (Modified and reprinted with permission from Ref. [36]. Copyright 2013)
Several molecular species in addition to methylene blue have been imaged using PPOCT. Phytochrome A, a naturally occurring molecular switch which may be reversibly optically pumped from one isomeric state to another, was imaged in a tissue phantom [37]. Hemoglobin was measured in the gill filament arteries of a zebrafish (Brachyrerio danio) using a time-domain 532 nm PPOCT system with a 532 nm pump [32]. The same system was also used to image the fluorescent protein DsRed in a transgenic zebrafish. Melanin was imaged in the first spectral-domain PPOCT system in a phantom made from human hair embedded in chicken breast tissue [38]. Melanin has also been imaged using a time-domain optical coherence microscopy system [39]. Recent work [36] has demonstrated volumetric imaging of microvasculature in Xenopus laevis using a two-color (532 nm pump, 830 nm probe) PPOCT system. Representative PPOCT cross sections overlain on the OCT cross sections are shown in Fig. 47.6 along with volumetric reconstructions of the vasculature measured with PPOCT. They have also demonstrated the use of the characteristic lifetime to differentiate PPOCT signals from two different chromophores.
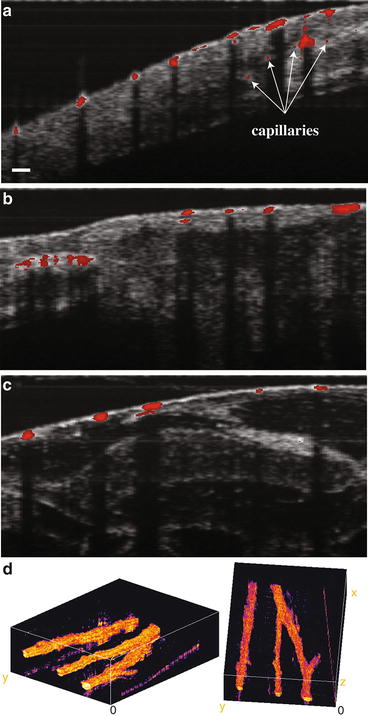
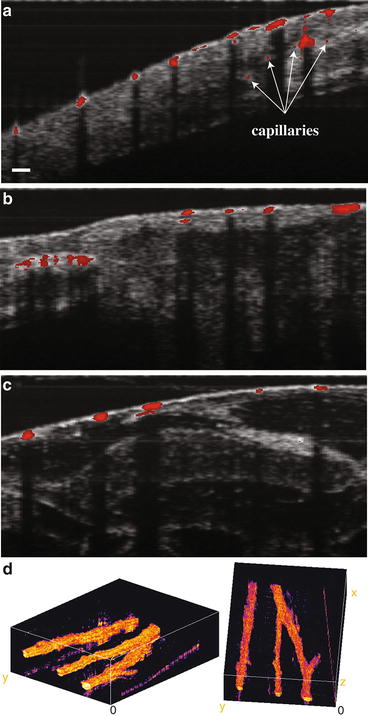
Fig. 47.6
(a–c) PPOCT B-scans overlaid on the corresponding co-registered OCT B-scans. Xenopus laevis vasculature is clearly depicted. Arrows in (a) point to capillaries that were not visible in conventional OCT. (d) Volumetric reconstructions of the microvasculature (Modified and reprinted with permission from Ref. [36]. Copyright 2013)
Figure 47.7 shows a pair of capillary tubes loaded with methylene blue/microspheres and bovine whole blood in heparin. The top panel (a) is the standard OCT image showing similar signal from both capillary tubes. A PPOCT image with 2 ns pump-probe delay (Fig. 47.7b) appears very similar to the OCT image. However, when the pump-probe delay is increased to 24.8 μs, the signal from the methylene blue/microsphere-loaded capillary tube decays, leaving only signals from the blood-filled capillary. Taking advantage of the difference in lifetime between two chromophores is an effective strategy for imaging multiple chromophores with PPOCT.
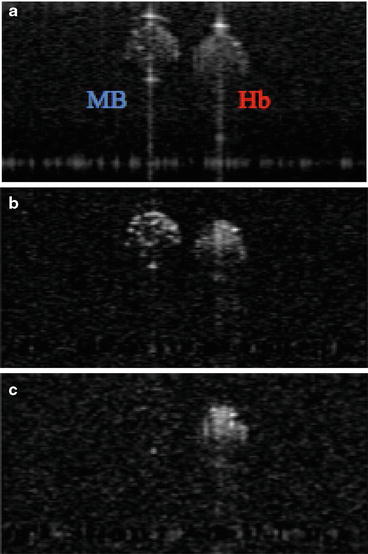
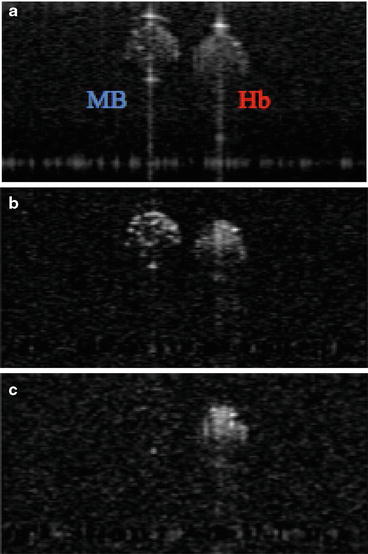
Fig. 47.7
(a) OCT B-scan of methylene blue (MB) and hemoglobin (Hb) in acrylic capillary tubes. (b) Corresponding PPOCT B-scans with a pump-probe delay of 3 ns. (d) Corresponding PPOCT B-scan with a pump-probe delay of 24.8 μs. The absence of the MB tube demonstrates the potential for using the lifetime as an effective method for differentiating multiple chromophores
Future research in PPOCT may lead in several directions. There is a clear potential for imaging vasculature. While Doppler-based OCT can also measure vasculature, a major advantage of PPOCT is that the signal is independent of the angle of flow, while the Doppler signal approaches zero when the illumination is orthogonal to the flow. The PPOCT signal is also molecularly specific, so it may be possible to differentiate oxy- and deoxyhemoglobin and develop a PPOCT-based measure of blood oxygen saturation. Furthermore, the imaging of exogenous contrast agents such as methylene blue could potentially be used to tag and image specific molecular species that are otherwise invisible to OCT. Such applications hinge on the demonstration of sufficient sensitivity either with methylene blue or some other discovered or engineered contrast agent.
47.5 Magnetomotive OCT
47.5.1 Theory and Instrumentation
Magnetomotive OCT (MMOCT) is a method for contrasting the distribution of magnetic particles based on their induced motion within a temporally modulated, magnetic field gradient [40]. Figure 47.8 illustrates the mechanism of MMOCT, showing how magnetic particles inside the imaging volume are mechanically pulled toward an electromagnet placed in the imaging arm of an OCT system. Typically, phase-sensitive OCT is then used to track the motion of light scattering tissue structures that are mechanically coupled to the particles [41]. Modulation of the electromagnet thus leads to phase modulation that can be band-pass filtered at the modulation frequency to detect the magnetomotion. Because human tissues are only very weakly magnetic (magnetic susceptibility |χ| < 10−5), MMOCT provides high specificity against the tissue background, on the order of 105 when using probes of χ ≈ 1 [42].
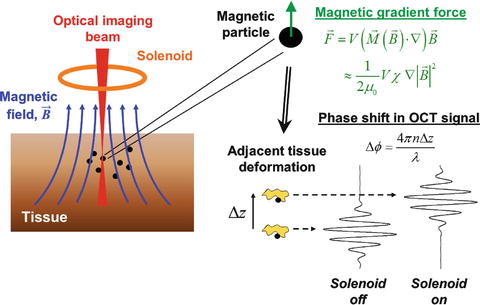
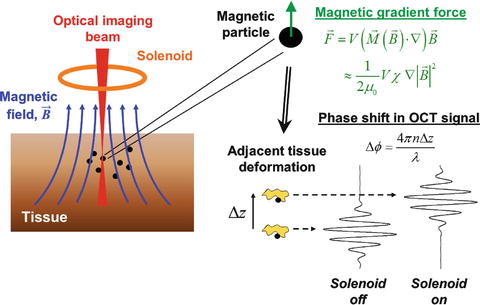
Fig. 47.8
Mechanism of magnetomotive contrast in OCT. A solenoid placed in the imaging arm of an OCT system provides a magnetic gradient force,
, on magnetic particles inside tissue according to the gradient of the magnetic field,
, and the magnetization and volume of the particles,
and V, respectively. The resultant elastic displacement of mechanically coupled light scattering structures, Δz, is sensed as a phase shift in the OCT interferogram, Δϕ. χ is the particle magnetic susceptibility, μ 0 is the vacuum permeability, and n is the tissue refractive index at the imaging beam wavelength, λ



A class of biomedical imaging probes currently used in MRI, called superparamagnetic iron oxides (SPIOs), are ideal for MMOCT because they are designed to exhibit large χ, avoid irreversible aggregation that is associated with ferromagnetic agents, and are composed of iron oxide which has a proven safety profile. FDA-approved MR liver contrast agents such as Feridex™, for example, have been shown to provide excellent MMOCT contrast [43]. Another type of MMOCT probe is protein microspheres encapsulating SPIO-containing ferrofluid, which then offer flexibility in adding targeting ligands and therapeutic payloads [44].
Implementing MMOCT on an existing phase-sensitive OCT system is relatively straightforward. A small electromagnet can be placed on either the same side (as shown in Fig. 47.8) or opposite side of the tissue to provide a magnetic field gradient oriented along the imaging axis. Somewhat counterintuitively, the strength of the magnetic field should only be on the order of 0.1T; higher fields will typically saturate the magnetic particles and reduce the detection sensitivity [41, 43]. The absolute sensitivity of MMOCT can be determined by considering the balance of forces between the diamagnetic tissue, which is pushed away from the magnet, and from the paramagnetic particles, which are pulled toward the magnet. For a typical SPIO particle, the minimum particle concentration needed to tip this force balance in favor of motion toward the magnet is on the order of 10 μg Fe/g. Another important consideration is the elastic property of the tissue medium. Magnetic particles in liquid do not undergo a restoring force during magnetic field modulation, moving only in one direction, and exhibit little contrast by conventional band-pass-filtered MMOCT. In a solid medium, the compliance of the tissue dictates the amount of displacement Δz, resulting in MMOCT contrast that is weighted by both the local particle concentration and the local tissue stiffness. Owing to the nanoscale displacement sensitivity afforded by phase-sensitive OCT systems, the tissue stiffness is typically of little detriment to the overall MMOCT sensitivity, and sensitivities as low as 27 μg Fe/g have been reported in optomechanical tissue phantoms [41]. The high sensitivity and specificity afforded by MMOCT have recently led to several new molecular imaging application areas, which will be reviewed below.
47.5.2 MMOCT of Atherosclerosis
Atherosclerosis is a disease in which an arterial vessel wall thickens as a result of the accumulation of fatty materials, including macrophages. Atherosclerosis is promoted by low-density lipoproteins (LDL) and cholesterol (crystals) and results in calcification in advanced lesions [45]. Standard intravascular OCT imaging has been extensively investigated for applications in cardiology such as imaging intraluminal 3D structure and function at high resolution, evaluating arterial stents, and visualizing atherosclerotic plaque [46–51]. The addition of contrast agents for use with intravascular OCT can enable site-specific molecular cardiovascular imaging, just as has been shown for ultrasound imaging using gas-filled microbubbles [52]. Targeted contrast agents may enable the early detection and localization of atherosclerotic lesions which may not be clearly evident in structural OCT imaging or in other imaging modalities. Therefore, the combination of intravascular OCT and targeted molecular contrast enhancement with MMOCT can potentially improve the sensitivity of early atherosclerotic lesion detection. Figure 47.9 shows representative MMOCT images from an ex vivo hyperlipidemic rabbit aorta. The RGD (arginine-glycine-aspartic acid)-functionalized protein microspheres [44] were fabricated to target the αVβ3 integrin overexpressed in atherosclerotic lesions [53]. These microspheres were loaded with SPIOs and a fluorescent dye, enabling multimodal imaging using MMOCT, MRI, ultrasound, and fluorescence imaging. The ex vivo aorta sample was perfused in a custom-designed flow chamber at physiologically relevant pulsatile flow rates and pressures. The functionalized microspheres have been successfully targeted to fatty streaks present during early-stage atherosclerosis. The future development of a MMOCT catheter or a new solenoid configuration for current commercial intravascular OCT systems may enable in vivo MMOCT imaging of atherosclerosis-targeted magnetic probes.
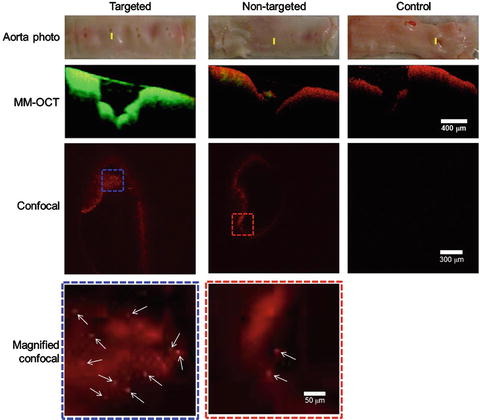
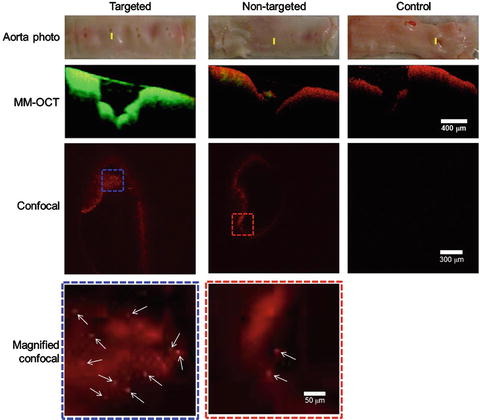
Fig. 47.9
Representative MMOCT and corresponding fluorescence confocal microscopy images of hyperlipidemic rabbit aortas after administration of RGD microspheres. Parametric MMOCT images are displayed showing the magnetomotive signal in green and the OCT signal in red. The MMOCT signal in the targeted microsphere group was statistically significantly higher (p < 0.01) than the nontargeted and control groups. Yellow lines in the aorta photos correspond to the imaging locations. The dotted blue and red boxes are magnified to show the presence of individual microspheres (white arrows). Scale bars are consistent across each row
47.5.3 MMOCT of Breast Cancer
Breast cancer is one of the most commonly occurring cancers in women and has a widespread effect on our society [54, 55]. Like other diseases, early detection is the key in the treatment of breast cancer. Breast cancer screening methods include manual clinical and self-breast exams, mammography, genetic screening, ultrasound, and MRI. Breast cancer cells often overexpress αVβ3 integrin and HER-2/neu receptors which are considered to be biomarkers for targeted cancer treatment [56]. Figure 47.10 shows an important step in breast cancer diagnosis using MMOCT to contrast HER-2/neu-targeted SPIOs [57]. In this study, the authors used a nitroso-methyl-urea (MNU) carcinogen-induced rat mammary tumor model. In vivo MMOCT images of tumors from rats injected with targeted SPIOs, nontargeted SPIOs, and saline exhibit an accumulation of SPIOs only in the tumors of rats injected with targeted MNPs.
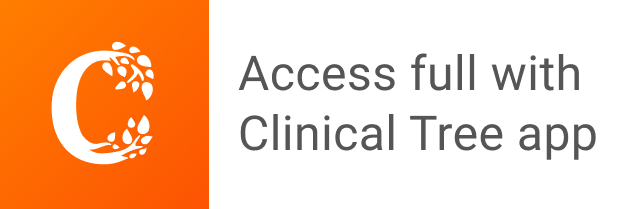