The principal advantage of the intraoperative HHOCT systems used to date in freehand mode is alignment versatility. A well-trained operator, normally the surgeon or an assistant, tilts and translates the probe, wrapped in a clear, sterile, plastic bag, steadily over the patient’s eye until good visibility of the retinal tissue is achieved. The freedom and range of tilting over the eye allows the surgeon to explore the retinal area and find the desired features quickly. The freedom of movement is particularly advantageous when the patient has reduced clarity through the pupil, such as with opacification of the lens capsule. The HHOCT used in microscope-mounted mode offers some stability advantage with foot pedal control over the freehand mode, however at the cost of alignment versatility.
The primary disadvantage of intraoperative HHOCT imaging is the unavoidable disruption to the procedure. The surgeon must remove any nonsecure surgical instruments from the eye and remove the operating microscope from the surgical field to bring the handheld probe into position.
Figure 58.2 illustrates HHOCT images taken in freehand mode immediately before and after surgical removal of the retinal ILM layer [25]. Postoperative morphology indicates reduced traction (tension), which is likely important for a favorable surgical outcome. The OCT images clearly demonstrate remnant ILM tissue. Under normal practices, membranes are stained to facilitate their visibility in the surgical microscope. OCT imaging can clearly delineate ILM and the underlying tissue when the ILM is peeled or elevated. Intraoperative HHOCT imaging also demonstrates small hemorrhages caused by instrument manipulation (Fig. 58.2(f)). This additional information may enable the surgeon to better assess whether the extent of peeling or other surgical maneuvers were adequate or whether additional maneuvers would be necessary.
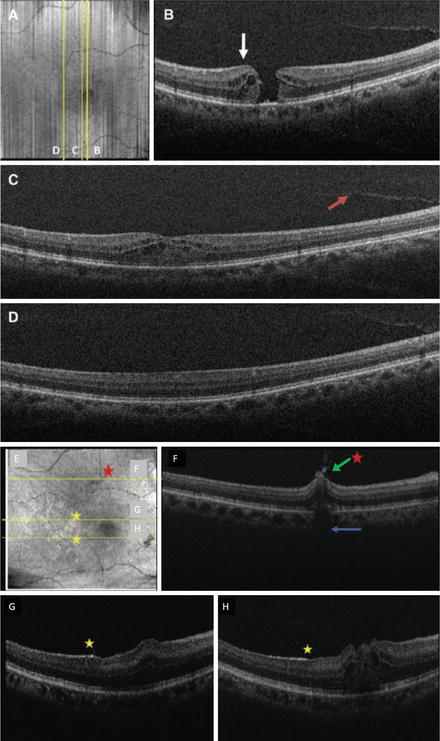
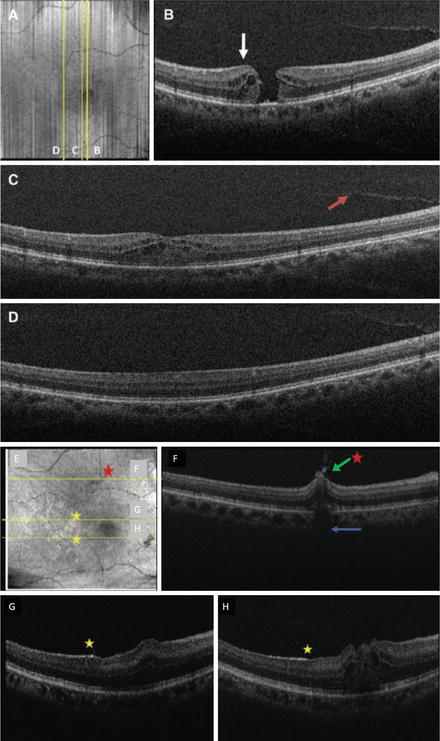
Fig. 58.2
HHOCT images of intraoperatively relevant features on a patient immediately before and after internal limiting membrane peeling [25]. In (a–d), images from an OCT volume dataset obtained pre-operatively are presented, while post-operative data obtained from the same location is shown in (e–h). (a, e) Surface volume projections of the volume datasets with yellow lines indicating positions of the individual B-scans shown following. In (b), cystic thickening adjacent to the macular hole is visible. In (c–d), partial vitreous separation is visible (red arrow). In (f), the green arrow indicates a pre-retinal hemorrhage corresponding to traumatic retinal hemorrhage caused by membrane peeling (red star). The yellow stars in (g–h) show remnant hyper-reflective ILM tissue
Because intrasurgical HHOCT is based on use of a commercially available product, it has so far likely been used by more investigators and on more patients than the more advanced but still experimental systems described in the next sections. The handheld approach has been shown to be effective at quickly evaluating the morphological changes of the target tissue at various stages during operations and has thus served as a test bed for future development of OCT guidance of intrasurgical procedures.
58.2.2 Microscope-Integrated Systems
To take full advantage of the potential for real-time OCT imaging during surgery and to minimize disruptions required for HHOCT imaging, several groups have reported on OCT systems integrated directly into the optics of the surgical microscope [32–51]. This approach has been termed microscope-mounted (MMOCT) and microscope-integrated (MIOCT) optical coherence tomography. We shall use the latter throughout this review because it better emphasizes the simultaneous operation of both the microscope and OCT systems. The underlying objective for an MIOCT system is to achieve en face and OCT imaging simultaneously without compromising the surgical view.
Integrated OCT designs take advantage of the optical components of the surgical microscope, which are optimized to achieve high-resolution en face imaging under a long working distance objective. This is suitable for manipulations on the surface of the eye, such as for anterior segment procedures. Visualizing the retina is achieved by cancelling or compensating for the power of the patient’s anterior optics, either by use of a flat outer surface lens in contact with the cornea or by reimaging the fundus to an intermediate plane with a positive ophthalmic lens. The latter approach is akin to indirect ophthalmoscopy. Multiple vendors have produced a range of contact lenses and versatile modules that mount the appropriate reimaging optics to the underside of the microscope.
MIOCT systems may combine the OCT beam into the visual path of the microscope either by division of aperture, such as through use of the assistant optical path [44, 45], or by division of wavefront, such as by use of a dichroic beamsplitter [32, 34, 36–43, 46–51]. To take advantage of the above-mentioned methods of viewing the desired field (of either cornea or macula), the OCT beam must optimally match the microscope’s normal vergence at the point of insertion, so that the OCT view matches and remains parfocal with the surgeon’s view.
A disadvantage of MIOCT systems is that they potentially increase the size, weight, and complexity of the surgical microscope suspended above the patient during surgery. For example, the prototype MIOCT system in current testing by our group at Duke University adds bulk which increases the height of the microscope body (from working lens to surgeon eye pieces). This is primarily due to the fact that these systems are currently experimental prototypes built as add-ons to commercial surgical microscopes. If preliminary testing by research groups is successful, microscope manufacturers could mitigate ergonomic issues by more fully integrating OCT in the design of future microscopes.
A photograph of the Duke prototype MIOCT system in use during surgery is shown in Fig. 58.3. Further details of MIOCT optical design and published results to date are provided below in Sects. 58.3 and 58.4, respectively.
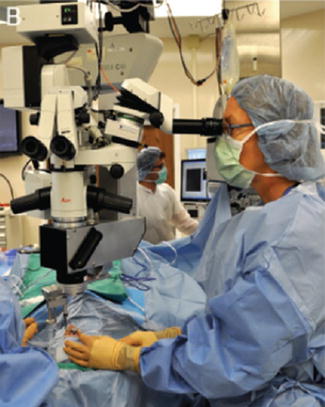
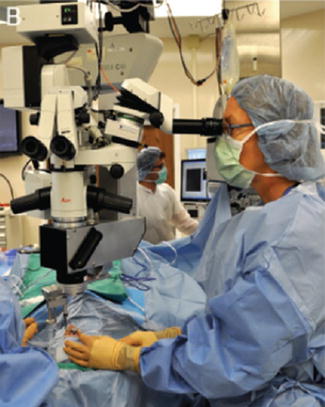
Fig. 58.3
The Duke prototype MIOCT system deployed in the ophthalmic operating room [42]. The surgeon’s eyepieces face the camera and the assistant is at the microscope
58.2.3 Intraocular Forward-Imaging Endoscopic OCT Probes
The development of forward-imaging OCT probes was first reported by Boppart et al. early in the history of OCT [52], but they were not initially targeted toward ophthalmic applications. More than a decade later, Han et al. [53] published work on an OCT scanning endoscope integrated into a narrow 21 gauge (810 um diameter) needle for retinal imaging. This design achieved excellent retinal image quality in animal eyes (Fig. 58.4). An unusual feature was the central wavelength of 1,310 nm, which is conventionally used for anterior segment but not retinal imaging because of strong absorption in the vitreous [54]. This issue was circumvented by the fact that the tip of the intraocular endoscopic probe was placed in close proximity to the tissue to be scanned. The working distance of the endoscope was 0.78 mm, leaving little room for vitreal absorption or for much error in hand movement.
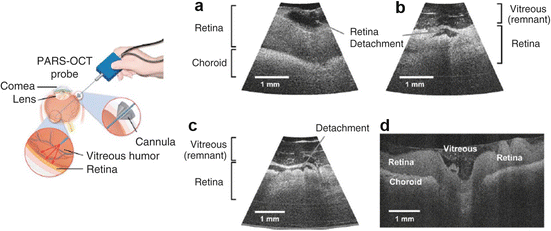
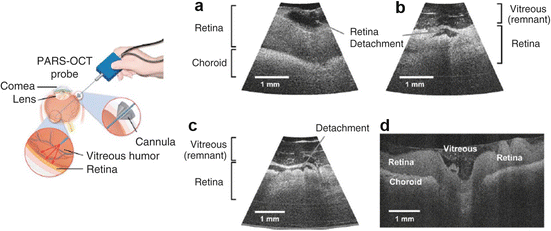
Fig. 58.4
Illustration of the forward-imaging endoscopic probe developed by Han et al. (left) and representative B-scans of porcine retinal tissue (right) [53]
One disadvantage of handheld endoscopes for intraocular surgery is the additional need it places on the surgeon for probe manipulation. Typically, one of the surgeon’s hands is used for holding an endoilluminator inside the ocular cavity to provide wide-angle illumination, while the second hand controls the tool that is actually manipulating the retinal tissue. Using endoscopic OCT imaging during tissue manipulations may therefore require the illumination to be fixed to a third insertion point in the sclera, such as in chandelier illumination.
Balicki et al. [55] reported an alternate intraocular probe design mounted inside a surgical needle. This effectively combined an OCT scanner with the manipulating surgical tool. However, the probe did not incorporate lateral scanning and thus only performed A-scans directly coaxial with the needle. Generating cross-sectional B-scans by sweeping the tool near the target tissue at a fixed distance by use of this probe was anticipated using robotic assistance, which may become prevalent in the future. This system also incorporated a fast ranging capability feedback to the motorized stage to compensate for patient motion by keeping the instrument tip at a fixed distance from the tissue.
To date, both of these forward-imaging ophthalmic probe systems have only been demonstrated on animal models.
58.3 Microscope-Integrated OCT System Design
In this section, we elaborate on the design and implementation details of MIOCT system hardware and control software, concentrating primarily on the system constructed and tested by our collaborative group at Duke University. We compare and contrast this design to those of other systems known to be under development, to the extent that details of these systems have been reported.
The Duke MIOCT system optical design and performance was first described by Tao et al. in 2010 [34] and the first human intrasurgical results (with the original custom-built OCT engine replaced by a commercial spectral-domain OCT console from Bioptigen, Inc.) were reported by Hahn et al. in 2012 [41, 42, 46]. An MIOCT system developed by Carl Zeiss Meditec (Jena, Germany) has been the subject of several reports by Binder et al. [32, 40]. A group at the University of Lubeck in Germany has developed and reported on a system which has been commercialized by OptoMedical Technologies GmBH (Lubeck, Germany) and Möller-Wedel (Wedel, Germany) [44, 45]. All three of these first-generation MIOCT systems share several similarities. All are based on spectrometer-based spectral-domain OCT engines operating near 850 nm wavelength, with coherence length (corresponding to axial resolution) of approximately 5 μm, and A-scan rates of 20–30 kHz. The principal distinction between first-generation systems appears to be in the sample arm scanner design and surgical microscope interface, which directly influences imaging performance. The Duke and Carl Zeiss systems are shared-objective designs utilizing the principle of division of wavefront, whereas the Möller-Wedel OCT design is based on division of aperture and occupies a separate collection path from the microscope visual optics.
The Duke MIOCT scanner optical design is illustrated in Fig. 58.5, along with a photograph of the scanner attached to a Leica M844 surgical microscope with an Oculus BIOM3 fundus viewing system. In this design, the OCT sample arm scanning beam is coupled into the infinity space directly above the microscope objective lens using a dichroic beamsplitter and aligned with the OCT optical axis midway between the stereo viewing path visual axes [34]. The OCT beam is shaped using a beam expander telescope prior to merging with the microscope visual path in order to achieve the standard lateral OCT resolution (∼15 μm) expected by retinal specialists. Thus, the OCT beam aperture in the shared region is larger than either of the stereo view optical apertures, with an associated reduction in depth of field compared to the visual stereo view. Optical design and test performance of the MIOCT unit are also illustrated in Fig. 58.5.
Insertion of the OCT scanning beam in the infinity space of the microscope view provides minimum interference with the operation of microscope components and accessories such as the zoom module, laser filters, camera ports, and assistant eyepieces, all of which are optically upstream from this position. Stacking of additional components in the infinity space does not alter the microscope resolution; however, care must be taken to avoid reducing the microscope field of view through vignetting, as well as to adjust the internal microscope illumination sources for extraocular and anterior segment work. The added height of the system (approximately 5 in. in the Duke system) can affect the posture of the surgeon and can be mitigated for shorter surgeons by using periscope oculars.
58.3.1 System Features for Managing the Challenging OR Environment
The Duke MIOCT scanner unit was designed as a surgical microscope accessory which is installed and aligned prior to surgery within approximately 10 min. The rest of the MIOCT system (the OCT “engine” and operating software) is a modified commercial spectral-domain OCT unit (from Bioptigen, Inc.) on a wheeled cart which is also brought into the operating room for MIOCT cases, similar to prior intraoperative OCT implementations [7]. All optical and electronic cabling between the OCT engine and the microscope-mounted scanner unit are suspended from the ceiling to avoid any tripping hazard.
Prior to use of the Duke MIOCT system in human surgical cases, extensive operating room evaluations were performed by having experienced anterior segment and retinal surgeons and surgical nurses interact with the MIOCT scanner unit in simulated surgeries. The participants were asked to evaluate how the scanner unit might interact with their normal surgical practices and maneuvers in a series of simulated tasks with defined evaluative assessments. The majority of responses identified no negative impact from having the device within their operating room environment. The overall impression was positive, and 100 % of surgeons and nurses reported that they would consider participating in human intraoperative MIOCT imaging trials [42]. Additional valuable feedback from these sessions, including recommendations on improved routing of the cables and rounding of the device edges, was also obtained to further improve the system.
58.3.2 Dynamic Navigation and Manual/Automated Tracking of Scanning Location
The principal advantage of microscope-integrated OCT scanning, in comparison to handheld systems described previously, is that microscope integration allows for the possibility of live image-based surgical guidance (as opposed to imaging during pauses in surgery). While current-generation clinical spectral-domain OCT systems routinely acquire B-scan images in real time, all available systems require at least several seconds for complete volume imaging of the macula. Even cutting-edge ultra-high speed swept-source OCT systems still cannot image the complete macula in real time in three dimensions with clinically acceptable optical power levels. A promising approach for matching the imaging rate capability of OCT with the real-time needs of surgical guidance is to implement a feedback-controlled tracking system to align the position of the live OCT B-scan (or a limited set of B-scans which can be acquired quickly) with the position on the macula which is of most immediate interest to the surgeon, such as the tip of the surgical tool in use.
Intraoperative OCT tracking has been implemented on the Duke MIOCT system using both fully automated and semiautomated “manual” tracking approaches (Fig. 58.6) [47–50]. In both approaches, a copy of the video feed from the surgical camera was used as the source of feedback to locate the position of the surgical tool tip (or other feature of interest to the surgeon), in order to direct the OCT scan location to that vicinity. In the automated approach, custom image processing software was developed to automatically locate the tool position and orientation in the video stream. While successful in phantom studies, this software is not yet capable of handling the wide range of illumination conditions in live human surgery. As an interim step, a semiautomated “manual” version of tracking has been implemented which takes advantage of a human observer who can accommodate varying surgical conditions and can also react to instructions from the surgeon. In manual tracking with the Duke MIOCT system, the live surgical view is displayed on a separate tracking computer in custom software operated by a human operator who tracks the mouse cursor to the position of current surgical interest on the macula. The mouse position is translated to a pair-offset voltage signals that are summed with the fast voltage signals controlling the MIOCT lateral scan position. The manual tracking feature has been found to enhance ease of use of the Duke MIOCT system in live human surgery, by improving OCT image placement accuracy and speed of alignment. This capability has led to faster imaging sessions and visualization of instrument-tissue interaction, which was not possible in HHOCT implementations.
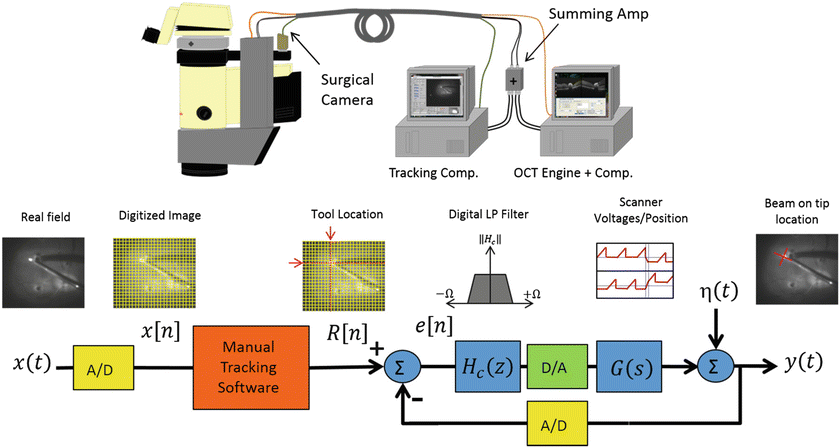
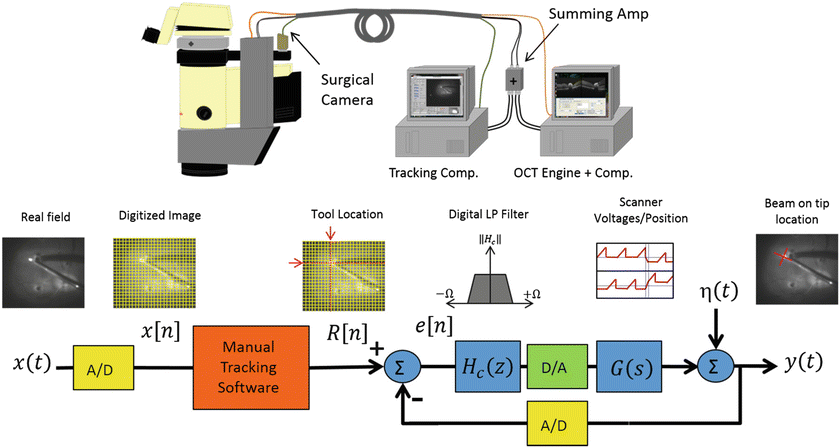
Fig. 58.6
The MIOCT tracking system being used in manual mode [50]. Hardware diagram of tracking and OCT computers (above), as well as the control system block diagram (below)
58.3.3 Scanning Orientation and Data Visualization
Since OCT volume datasets are acquired quickly in the B-scan direction, and then more slowly in the azimuthal direction, it can be important to choose the scanning direction based on prior knowledge of object motion in the scanning region. For intraoperative OCT imaging, there are many possibilities to orient scanning axes relative to tissue features and/or instrument position and trajectory. As an illustration, in Fig. 58.7, a series of OCT B-scans scans were taken both perpendicular and parallel to a common vitreoretinal surgical tool, the Tano Diamond Dusted Membrane ScraperTM [43]. These images were taken in cadaveric porcine eyes. The relative transmission and shadowing of the tool can be clearly seen, and the contact between the instrument and tissue can be examined easily. For tracking maneuvers, such an orthogonal scan pattern comprised of rapidly sequentially acquired orthogonal scans with their intersection locked to the tool position and their orientation matching the tool axis may be advantageous. Alternative patterns such as a fixed scan on morphologic features may also be desired.
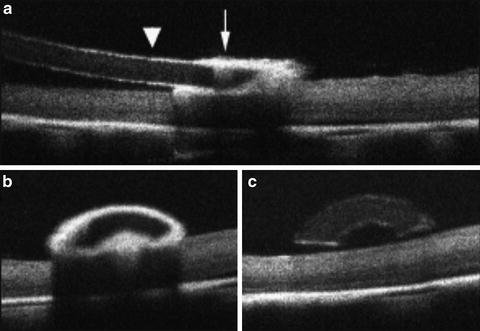
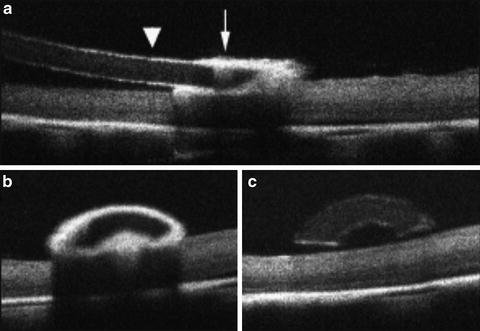
Fig. 58.7
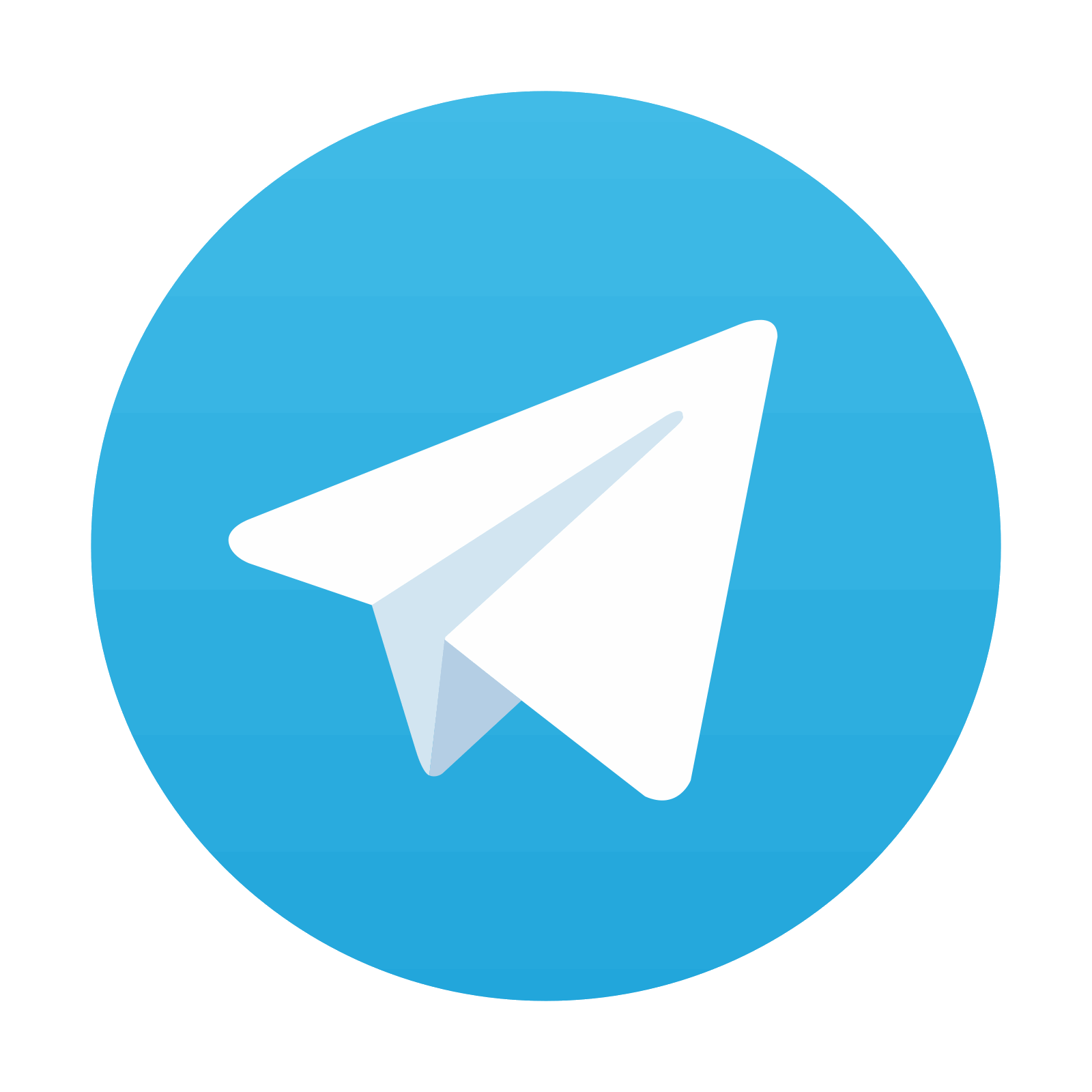
Averaged B-scans of a surgical instrument in contact with a cadaveric porcine retina. (a) The view along the instrument axis. (b) and (c) are perpendicular views of the instrument from the locations at the diamond-dusted tool tip and along the instrument shaft, respectively. The highly scattering diamond-dusted section creates considerable shadowing over the retina, while the silicone shaft creates relatively little
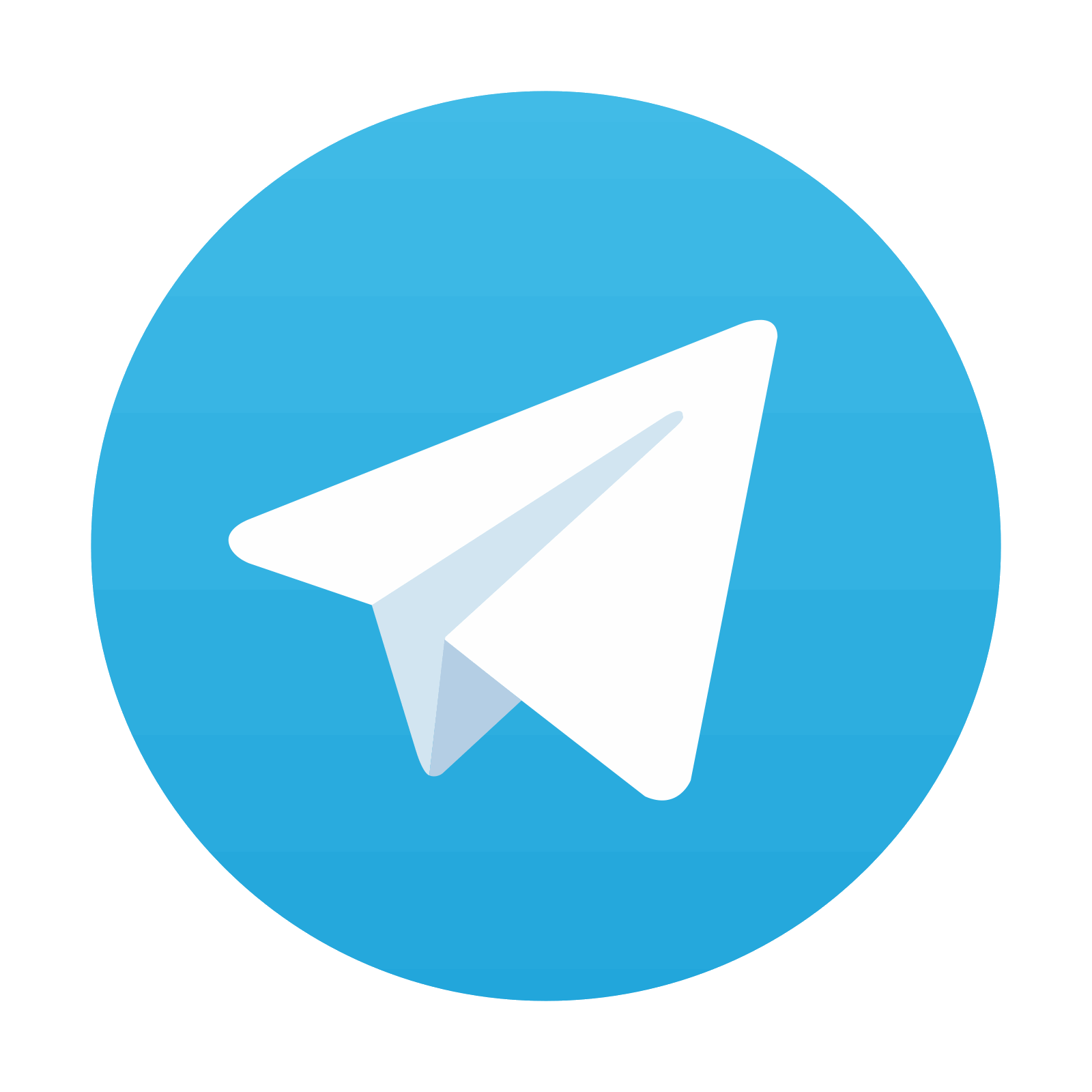
Stay updated, free articles. Join our Telegram channel
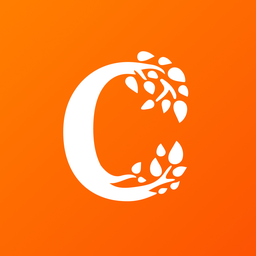
Full access? Get Clinical Tree
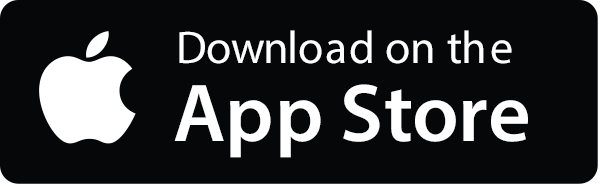
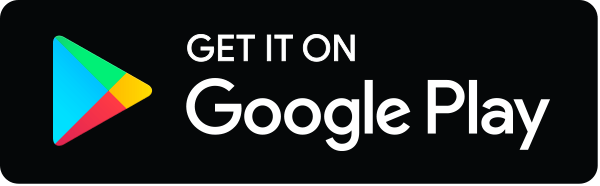
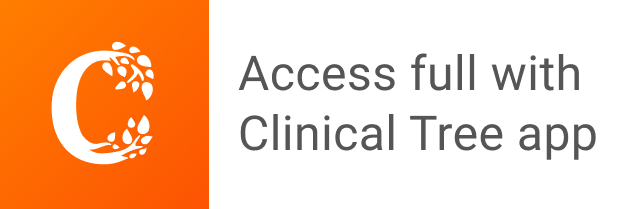