Fig. 51.1
Multi-scale biomedical imaging modalities and platforms for multimodal imaging (indicated by “⇔”). PET positron-emission tomography, SPECT single-photon emission computerized tomography, MRI magnetic resonance imaging, X-ray CT X-ray computerized tomography, DOT diffuse optical tomography, US ultrasound, PAT photoacoustic tomography, OCT optical coherence tomography, LOT laminar optical tomography, CM confocal microscopy, and MPM multiphoton microscopy (Reproduced from Ref. [20] with permission © 2010 IEEE)
51.2 Combined OCT and FLOT: Instrumentation
A co-registered OCT and line-scan FLOT system was developed in our lab [19, 20]. Figure 51.2 shows the schematic of the multimodal imaging system. The OCT subsystem utilized a wavelength-swept laser as the light source with a spectrum centered at 1,310 nm and bandwidth of 100 nm, thereby providing an axial resolution of 10 μm in the tissue. The laser operated at a sweeping rate of 16 K A-scan/s with an average output power of 12 mW. The output of the swept laser was split into two paths: 3 % was sent to a Mach–Zehnder interferometer (MZI); the remaining 97 % was equally distributed to the sample and reference arms of the OCT Michelson interferometer, which was composed of one circulator and one fiber-optic 50/50 splitter. The light in the sample arm passed through a collimator (C), a pair of galvanometer mirrors (X and Y), and an objective to the tissue. The power on the tissue was 4 mW with a spot size of 15 μm. The reference arm consisted of a dispersion compensation glass (DCG) and a polarization controller (PC). DCG matched the dispersion induced by the optics in the sample arm. The light returned from the sample and reference arms formed the interference signal, which was electronically detected by a balanced detector (BD). The output of BD was discretely sampled by a data acquisition board (DAQ, AlazarTech), which was triggered by the zero-crossings of the MZI fringes. The zero-crossings provided an equally spaced frequency clock, therefore eliminating the need to interpolate/resample the discrete interference signal and increase the speed of data processing. Lastly, discrete Fourier transform rendered the axial profile of the tissue (A-scan) with 2.5 mm imaging depth in 512 pixels. The sensitivity (the minimal detected signal) of the OCT subsystem was 95 dB.
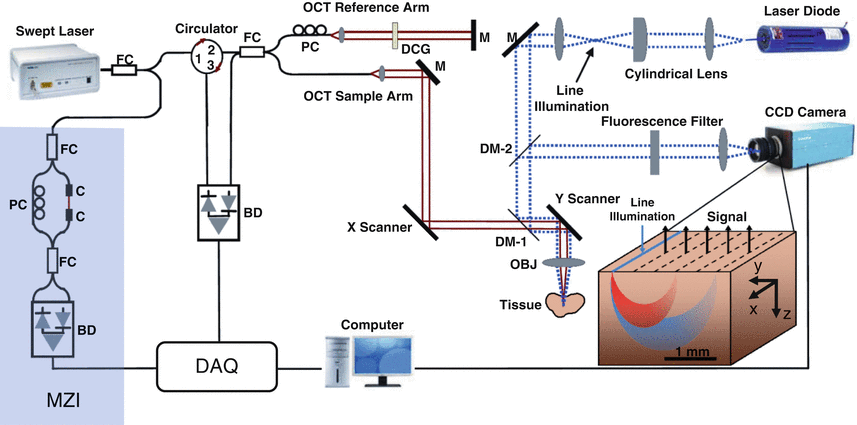
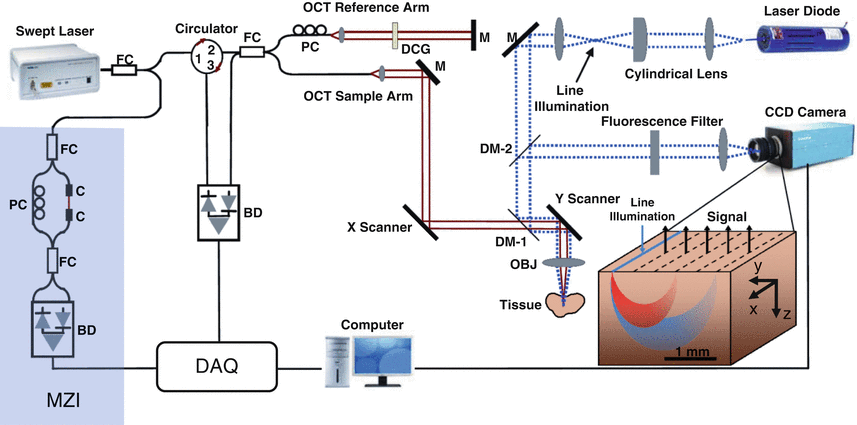
Fig. 51.2
Schematic of the combined OCT and line-scan FLOT system (Reproduced from Ref. [20] with permission © 2010 IEEE)
The line-scan FLOT subsystem is shown in the right portion of Fig. 51.2. The excitation source was a continuous wave (CW) laser diode at 670 nm. A cylindrical lens was used to expand the illumination point to a line. Line illumination simplified the scanning mechanism by eliminating the scanning along the illumination dimension. The excitation line sequentially passed through a dichroic mirror (DM-2), galvanometer Y, and another dichroic mirror (DM-1) before illuminating on the tissue. The line illuminated on the tissue was 2.2 mm long in x-direction and 25 μm wide in y-direction. The illumination power was 8 mW. DM-1 integrated the FLOT optical axis and the OCT sample arm. Specifically, DM-1 transmitted the OCT light (at 1,300 nm) and reflected both the FLOT excitation and emission light (<850 nm). DM-2 further separated the excitation reflectance and the fluorescence emission light. The fluorescence light passed a band-pass filter (700 ± 10 nm) to the electron-multiplying CCD (EM-CCD, Cooke Corp.). EM-CCD served as a detector array with one-dimension (orthogonal to the line illumination) pixels collecting the lights with different separations from the illumination spot.
The total acquisition time of this OCT–FLOT co-registered system is 10 s for each 3D volume. For OCT, the beam was scanned in two axes. The fast scanning in x-direction was set at the speed of 25 Hz (x-scan was 2.5 mm wide in 624 pixels), and the slow scanning in y-direction was set at the speed of 0.1 Hz (y-scan was 2.5 mm wide in 256 pixels). For line-scanned FLOT, only scanning in y-direction was needed, therefore the scanning speed was the same as OCT y-scan. EM-CCD totally acquired 256 frames, each of which was exposed 30 ms. The resulting OCT 3D volume size is 624 (x) by 256 (y) by 512 (z) pixels (with voxel size of 4 μm × 10 μm × 4.4 μm, and FLOT image raw data is 501 (x) by 1,024 (y) (2.2 mm × 2.2 mm) by 256 frames. The rate-limiting factors were the FLOT CCD frame rate and the high number of OCT images acquired in the y-dimension. This 10 s acquisition time can be reduced by binning the EM-CCD detector arrays and/or reducing the camera field of view (FOV). If focusing on 2D (xz) cross-section imaging (B-scan) only, it shall be possible to perform real-time FLOT imaging by reading only partial regions of the EM-CCD.
51.3 FLOT Theory and Image Reconstruction
Depth-resolved FLOT image is obtained through image reconstruction process similar to that used in DOT [21]. The fluorescence signal
can be expressed as [17, 22]:

where σex(m2) is the absorption cross section of the fluorophores at the excitation wavelength, γ(unitless) is the spectral quantum yield of the fluorophore,
is the excitation fluence distribution calculated from the excitation photon radiance from a point source located at
and at the angle
is the probability density that a photon emitted by a source at position
will be detected by a detector located at
and at the angle
, and
is the fluorophore concentration at position
. Equation 51.1 can be discretized into voxels, which yields a matrix equation:

where F is the fluorescence measurements, C is the spatially distributed fluorophore concentration, and J is the sensitivity matrix that represents the spatial dependence of each measurement to the fluorophore concentration at each voxel. J is expressed as



(51.1)









(51.2)

(51.3)
The sensitivity distribution of FLOT can be calculated using Monte Carlo simulation (see Fig. 51.3). The sensitivity profile varies as the source–detector separation increases as well as the incidence/detection angle. Therefore, depth-dependent information is encoded in the multiple detector measurement and can be recovered through image reconstruction.
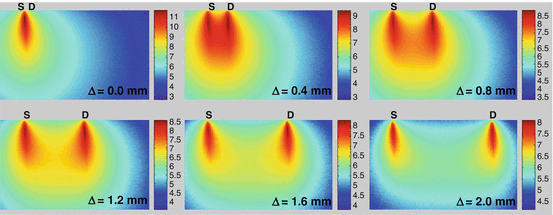
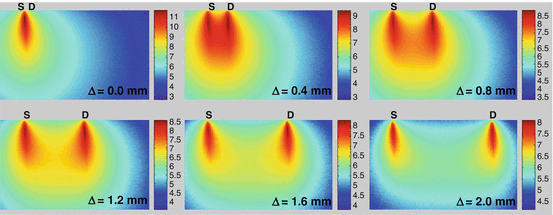
Fig. 51.3
Monte Carlo simulated measurement sensitivity distribution of FLOT measurements (log scale). Tissue geometry was 3 mm (lateral) by 2 mm (depth) with scattering coefficient μ s = 8 mm−1 for excitation and 7 mm−1 for emission (g = 0.9) (Reproduced from Ref. [20] with permission © 2010 IEEE)
Image reconstruction was performed within individual plane (YZ) perpendicular to the illumination line (X). Two main approaches were implemented. First, simultaneous iterative reconstruction technique (SIRT) [23] was used in our phantom experiments and subsurface cancer imaging (Sect. 51.4). The nonnegativity of
posts a hard constraint in the reconstruction; in other words, the negative elements will be replaced to zeros after each iteration. Iterations stopped until the change between iterations was <0.1 %. Second, the least square fitting with Tikhonov regularization [11] was used in the engineered tissue imaging (Sect. 51.6). The Tikhonov approach can be expressed as
![$$ C={\left[{J}^TJ+{\uplambda}^2{S}_{\mathrm{Max}}^2I\right]}^{-1}{J}^T\mathrm{F} $$](/wp-content/uploads/2017/03/A76297_2_En_52_Chapter_Equ4.gif)
where SMax is the largest singular value in J, superscript T stands for transpose operation, and λ is the normalized regularization factor that leverages the signal level and noise level. The criteria of discrepancy principle were implemented to determine λ, which was typically 3 × 10−4. Because of explicit inversion, the time of the Tikhonov approach was faster than the SIRT approach. (0.06 s vs. 2 s).

![$$ C={\left[{J}^TJ+{\uplambda}^2{S}_{\mathrm{Max}}^2I\right]}^{-1}{J}^T\mathrm{F} $$](/wp-content/uploads/2017/03/A76297_2_En_52_Chapter_Equ4.gif)
(51.4)
51.4 Results: Phantom Experiments and Subsurface Cancer Imaging
The performance of the OCT–FLOT system was first demonstrated by imaging a tissue phantom, which consisted of a capillary tube with an inner diameter of 110 μm and an outer diameter of 130 μm, suspended in a scattering medium consisting of 2 % Intralipid mixed with Indian ink μ a = 0.2 mm−1, μ s = 7.2 mm−1 at 670 nm, and anisotropy factor g = 0.9, similar to those of biological tissues. The tube was filled with 10 μM fluorescent dye Cy5.5.
Figure 51.4 shows the comparison of OCT and FLOT images of a capillary tube phantom. OCT readily images the 3D structure of the tube as shown in Fig. 51.4a. Figure 51.4b shows the comparison of OCT cross-sectional image (YZ) and FLOT image at location “1” denoted in (A). Cross-sectional OCT revealed the capillary tube (indicated by the arrow) with approximately 0.6 mm beneath the surface. FLOT cross-sectional image showed a fluorescence object at the same location, with a slightly larger size due to the relatively larger FLOT resolution compared to OCT. Cross-sectional OCT and FLOT images at location “2” also showed good agreement with the tube depth of approximately 0.9 mm (Fig. 51.4c). Compared to (B), the FLOT image for deeper object showed slight enlargement in size. In addition, the peak values were slightly reduced when the object went deeper due to the enlargement of the reconstructed object size. Figure 51.4d shows the OCT cross-sectional image along the tube’s longitudinal dimension (XZ). The tube was placed at an angle with respect to the horizontal axis, and slightly bending flat. The FLOT image (XZ) revealed similar contour of the capillary tube as shown by OCT. Also, this image was averaged over a range of 150 μm in y-dimension across the central axis of the tube; therefore, the results indicated nearly constant fluorophore concentration. Here OCT provided the structural information of the phantom, and the FLOT reconstructed image provides the fluorescence dye distribution information. In biomedical applications, FLOT would provide fluorescence-dye-targeted molecular information. Together, the hybrid OCT–FLOT system can be used for concurrent depth-resolved tissue structural and molecular imaging.
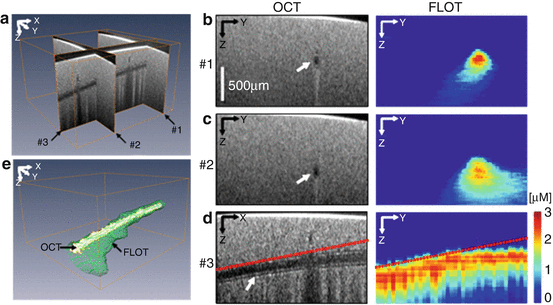
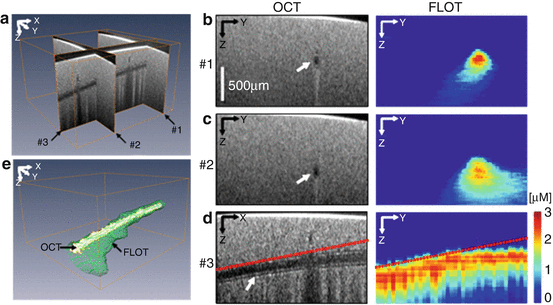
Fig. 51.4
Co-registration of OCT and line-scan FLOT of a capillary tube filled with fluorescence dye. (a) 3D OCT imaging showing three representative slices. (b) Left, OCT image (slice #1) of the capillary tube (arrow); right, FLOT image reconstruction of the fluorescence object. (c) OCT and FLOT images of different position (slice #2). (d) OCT and FLOT image (slice #3) reveal the curvature of the capillary tube (two identical dotted lines serve as the reference slopes). (e) 3D isosurface of FLOT image showed good co-localization with OCT (Reproduced from Ref. [19] with permission © 2009 OSA)
Using the same system, an animal model of breast cancer was imaged [20]. A triple negative human breast cancer cell line, MDA-MB-231, originally expanded from a pleural effusion, was obtained from the American Type Culture Collection (ATCC). Plasmid DNA encoding tdTomato (pRSETB-tdTomato) was cloned into the mammalian expression vector pEF-1α-myc/his (Invitrogen, Carlsbad, CA) generating pEF-1α-tdTomato. pEF-1α-tdTomato was stably transfected into MDA-MB-231 cells using the Amaxa Nucleofector II instrument (Amaxa Biosystems, Gaithersburg, MD), followed by fluorescence-activated cell sorting (FACS) of tdTomato-fluorescing cells, and further expansion without antibiotics. Prior to inoculation, MDA-MB-231-tdTomato cells displayed intense tdTomato fluorescence in cell culture as tested with live cell fluorescence microscopy [24].
One hundred thousand to two million MDA-MB-231-tdTomato cells were orthotopically inoculated in the mammary fat pad of anesthetized female athymic nude mice as previously described [25]. Tumor xenografts reached their final experimental size of about 5 mm3 within 4 weeks. In vivo whole-body fluorescence imaging of MDA-MB-231-tdTomato tumor xenografts was confirmed using a commercially available optical imaging system, the Xenogen IVIS 200 Spectrum (Caliper Life Sciences, Hopkinton, MA) before in vivo OCT–FLOT imaging under anesthesia.
Figure 51.5 shows OCT–FLOT imaging of human breast cancer xenograft model in vivo. Figure 51.5a shows an OCT cross-sectional image of the breast tumor. The high scattering mouse skin layer limited the penetration depth. However, the boundary between the skin and the tumor remained visible (arrow). Figure 51.5b shows the co-registered FLOT image revealing the subcutaneous tumor (which was transfected with red fluorescence protein). Figure 51.5c is the fused OCT–FLOT image which shows the relative position and distribution of tumor regions underneath the skin. Figure 51.5d shows the corresponding histology confirming the presence of tumor. Figure 51.5e shows the OCT cross-sectional image of another region of the breast tumor. The boundary between skin and tumor was less well defined (arrow) indicating tumor invasion. Figure 51.5f shows the co-registered FLOT image revealing a larger tumor volume than that shown in (B). Figure 51.5g shows the fused OCT–FLOT image. The corresponding histology (h) confirms the larger tumor size than (d). Assuming oval-shaped tumor, FHWM of the long and short axes of the tumor were 2.17 × 0.92 mm2 for the smaller tumor region (b) and 2.50 × 1.11 mm2 for the larger tumor region (f). The histology findings indicated that the tumor sizes were 2.30 × 0.83 mm2 and 2.33 × 1.25 mm2, respectively (d, h). These results indicated that FLOT can visualize the difference in subsurface tumor sizes. Figure 51.5i shows the 3D view of mouse skin (from OCT) and subsurface tumor (from FLOT). This result demonstrates the feasibility of simultaneous morphological and molecular imaging of subsurface tumor in mesoscopic scale using OCT–FLOT.
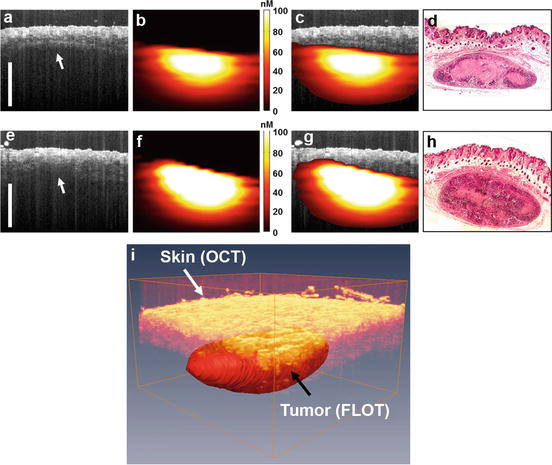
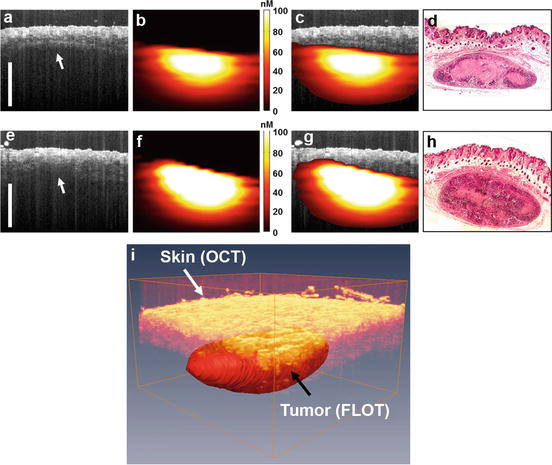
Fig. 51.5
OCT–FLOT of subcutaneous human breast tumor xenograft on mouse model in vivo. Breast cancer cells (MDA-MB-231) were constitutively labeled with tdTomato red fluorescence protein. (a) OCT cross-sectional image of breast tumor. Mouse skin layer shows high scattering, and the boundary between skin and tumor is clearly visible (arrow). (b) Co-registered FLOT image revealing subsurface tumor. (c) Fused OCT–FLOT image and (d) the corresponding histology. (e) OCT cross-sectional image of another region of the breast tumor. The boundary between skin and tumor was less well defined (arrow). (f) Co-registered FLOT image revealed a larger tumor volume than (b). (g) Fused OCT–FLOT image. The corresponding histology (h) confirmed the larger tumor size than (d). (i) Subcutaneous breast tumor xenograft in vivo was rendered in 3D. Tumor cells were transfected with red fluorescence protein (RFP) and displayed as isosurface of 30 nM of calibration dye Rhodamine 6G. Tumor size: 2.2 × 2.3 × 0.9 mm3. Bar: 1 mm (Reproduced from Ref. [20] with permission © 2010 IEEE)
51.5 Improvement of FLOT Resolution Using Angled Configuration
One of the limitations for our current FLOT system is that the point spread function (PSF) enlarges when the object locates deeper [11, 20]. Our previous measurement (see Fig. 51.4e) indicated that the axial PSF was ∼100 to 200 μm at ∼0.6 mm depth and increased to ∼300 to 400 μm at ∼1 mm depth [20]. To overcome this limitation, we investigated the effects of source and detector angles on the imaging performance [26]. Previous work in optical spectroscopy suggests that using angled illumination–collection design (oblique illumination–collection) will enhance the depth selectivity of epithelium tissues [27–31]. The angular degree of freedom in the illumination and detector arms is investigated first using the theoretical analysis and simulation. Then we implemented this design and experimentally demonstrated that angled FLOT (aFLOT) increased the depth selectivity and resolution.
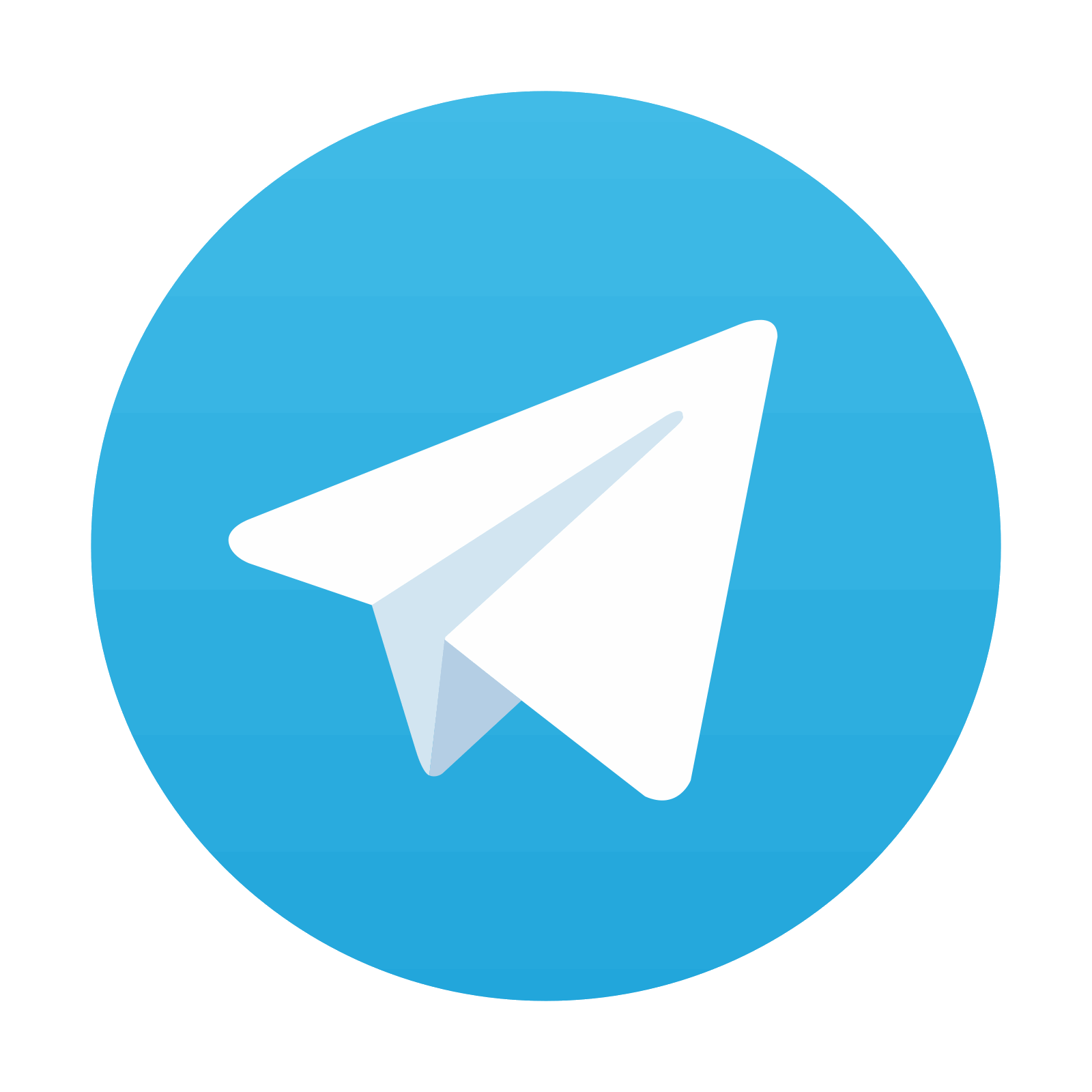
Stay updated, free articles. Join our Telegram channel
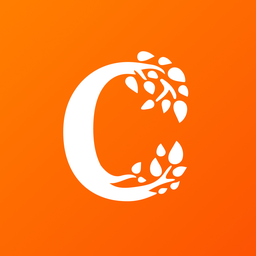
Full access? Get Clinical Tree
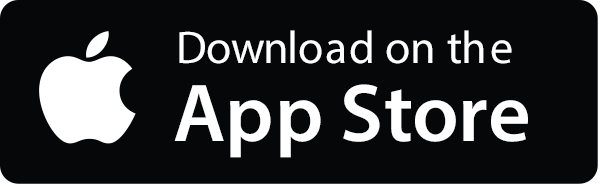
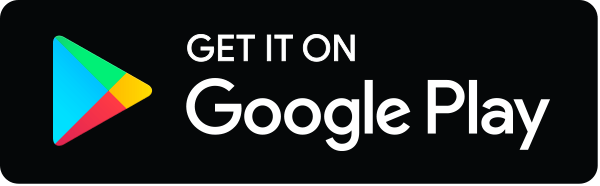