CHAPTER 1 Genetics and Otolaryngology
Genetics plays a role in just about everything, although the magnitude of that role may be, in a few cases, barely detectable. It is important—and quickly becoming even more so—for the otolaryngologist to understand genetics to carry out his or her medical obligations effectively. There are hundreds of syndromes that affect the head and neck,1 and people with most of these syndromes come under the care of an ear, nose, and throat (ENT) surgeon at one time or another during their lives. Furthermore, hearing is an important aspect of the otolaryngologist’s practice, and more than 50 different genes have been identified that cause nonsyndromic hearing loss (see the Hereditary Hearing Loss home page at http://webh01.ua.ac.be/hhh/). The identification of the basic dysfunction underlying all of these single-gene disorders is the first step to effective prevention and treatment, whether surgical or pharmacologic. An understanding of complex (presumably polygenic) disorders has become an extremely important objective for medical research. Liability for a variety of diseases (e.g., head and neck cancer, otosclerosis, otitis media, dyslexia) is controlled by several genes.2–5 It is thought that the day is near when knowledge about an individual’s genotype will identify those who are at high risk for development of these and other disorders.6
The Genome
The term genome refers to the collection of all of the genes that an organism possesses. It has been estimated that there are between 40,000 and 140,000 genes in the human genome. The genes are assembled into lengthy strands of deoxyribonucleic acid (DNA), which are organized linearly into chromosomes. The chromosomes are made up of the DNA that forms the genes and the intervening DNA as well as chromatin, which is a protein that assists in the maintenance of the structure and regulation of chromosomal expression. The nuclei of most human cells contain 46 chromosomes that are organized as 23 pairs. Except for the mitochondria, all genes that are contained within the human genome lie on one or the other of these chromosomes. The linear order of the genes on the chromosomes allows one to create maps of the human gene order; these maps are generally invariant throughout any given species. It is these maps that allow us to associate specific genes with specific traits through gene mapping.7,8 Genes are transmitted in groups that correspond with the chromosomes. Aside from cases of the exception known as “crossing over,” all of the genes of one chromosome (e.g., the paternal one) are transmitted together to the exclusion of the other one. Crossing over allows one chromosome to become a mosaic of both paternal and maternal genes; the frequency with which crossing over occurs has been studied and forms the basis for one of the two ways of measuring distances between genes. The physical distance between two genes is the number of bases between genes and is measured in megabases or kilobases. The genetic distance between two genes, however, is based on the frequency of observed recombination between them and can only be estimated by the study of informative matings and their offspring. Genetic distance is the result of a biologic phenomenon and is imperfectly correlated with physical distance. The order of genes on a chromosome is constant.
Unfortunately, the information in the genome is simply not organized into rational groupings. One purpose of the genome project had been to develop an index of the genome that would allow researchers who are trying to connect specific genes with specific disorders to do so efficiently.9,10 From the perspective of the otolaryngologist, this means first that gene-specific diagnoses are now available for many ENT-related disorders and that ultimately better therapies will emerge as more is learned about the basic nature of hereditary disorders that affect hearing, speech, and the structures of the head and neck.
The genome revolution has placed mankind at the brink of the development of new and exciting therapies for both rare and common genetic disorders.11 It will thus become increasingly important for the ENT practitioner to recognize those disorders that have a strong genetic component.
Gene Structure and Expression
The basic eukaryotic gene is made up of exons and introns. Exons make up the coding part of the gene; the intron is DNA that is interspersed between the exons. During the process of creating a message, introns are spliced out of the message, thus leaving only the exons to be translated into protein. The gene is thus an interrupted sequence of code that must be further processed into a usable message. Figure 1-1 illustrates how the structure of the gene is related to the process of transcription. During transcription, the whole gene is copied—exons and introns together—into a premessage. The premessage is then processed by excising the introns and joining the exons together to make a series of bases that code for a protein. The sites at which the excisions and rejoining take place are called splice sites, and specific sequences of bases are used to signal the cellular machinery to recognize these places. There are specific start and end points for the transcription of the gene as well; within the genes, there are specific signals in the form of three-base sequences (the start and stop codons) that indicate where translation into protein is to begin and end. The gene is thus made up of a coding sequence and punctuation.
Chromosomal Disorders
With some exceptions, chromosomal disorders are generally not heritable. Physical abnormalities associated with chromosomal imbalance are the result of rather extensive duplications or deletions of genetic material and involve multiple genes. The most common chromosomal disorder that involves the autosomes is trisomy 21.12 Chromosomal disorders can be classified into one of four groups:
Aneuploidies
A trisomy is demonstrated when three copies of a whole chromosome occur in an offspring. This happens because of nondisjunction, which is the movement of a pair of chromosomes to the same pole during cell division; this results in one daughter cell lacking that chromosome and the other daughter cell possessing an extra copy of that chromosome.13 The three major autosomal trisomies are 21, 18, and 13.
Trisomies 13 and 18 are not compatible with long life, and the average life span of individuals with either of these conditions is less than 1 year. The majority of infants with trisomy 13 are profoundly deaf and have a cleft lip and palate in addition to multiple other congenital anomalies.14,15 Hearing loss is frequent in trisomy 18 as well.16 However, hearing and head and neck anomalies are unlikely to be a serious concern because of the limited survival.
Patients with trisomy 21 have ears that are smaller than normal. About 75% have hearing loss, which can be sensorineural, conductive, or mixed.17 The prognosis of a child with trisomy 21 is generally good, and correction to normal hearing is important for helping such a child achieve maximal abilities.18
The common aneuploidies that involve the sex chromosomes include 45,X (Turner’s syndrome, phenotypic female) and 47,XXY (Klinefelter’s syndrome, phenotypic male). Although profound hearing loss is infrequent, mild to moderate hearing loss is common in 45,X individuals.19–21 Females with Turner’s syndrome are highly susceptible to otitis media, but whether this changes with hormone replacement therapy remains to be investigated.20,22,23 About 25% of children with Klinefelter’s syndrome have a mild sensorineural hearing loss.24,25 Hearing losses in both Turner’s and Klinefelter’s syndromes often remain undetected.
Single-Gene Disorders
The terms dominant and recessive usually refer to the pattern of inheritance of a particular disorder, but, more importantly, they communicate the way in which combinations of two alleles produce a specific (usually abnormal) phenotype. With a dominant inheritance pattern, individuals who carry one copy of the mutant allele (heterozygote) or two copies of the mutant allele (homozygote) are equally affected. With recessive inheritance, a person must be homozygous for the mutant alleles; individuals who are heterozygous are normal. When using these terms to describe a disease, if the disease is called dominant, then normal is recessive, and vice versa. True dominance is probably uncommon. Branchio-oto-renal syndrome (BOR) is described as dominant,26 but because it is uncommon a true mutant homozygous individual has probably never been born. Most geneticists would expect that patients with the homozygous mutant form of BOR would have a more severe phenotype, one that is possibly even lethal. Similarly, one might expect that patients with many of the recessive nonsyndromic deafness disorders could have a mild manifestation in the heterozygote, possibly contributing to the liability of the development of age-related hearing loss. An example of true dominance occurs with Huntington’s disease, in which a homozygous patient who is affected has the same phenotype as heterozygous individuals.27,28
Dominant Disorders
Figure 1-2 shows a typical family pedigree of an autosomal dominant disorder. Under full penetrance, each affected individual has an affected parent. Because they are heterozygous, each affected individual has a 50% chance of transmitting the abnormal gene to offspring, each of whom would be similarly affected. The only reasonable instance in which a person could be homozygous and affected would be if both parents were affected. Dominant mutations are recognized through their pattern of inheritance, which typically shows vertical transmission and the involvement of several generations and several sibships.
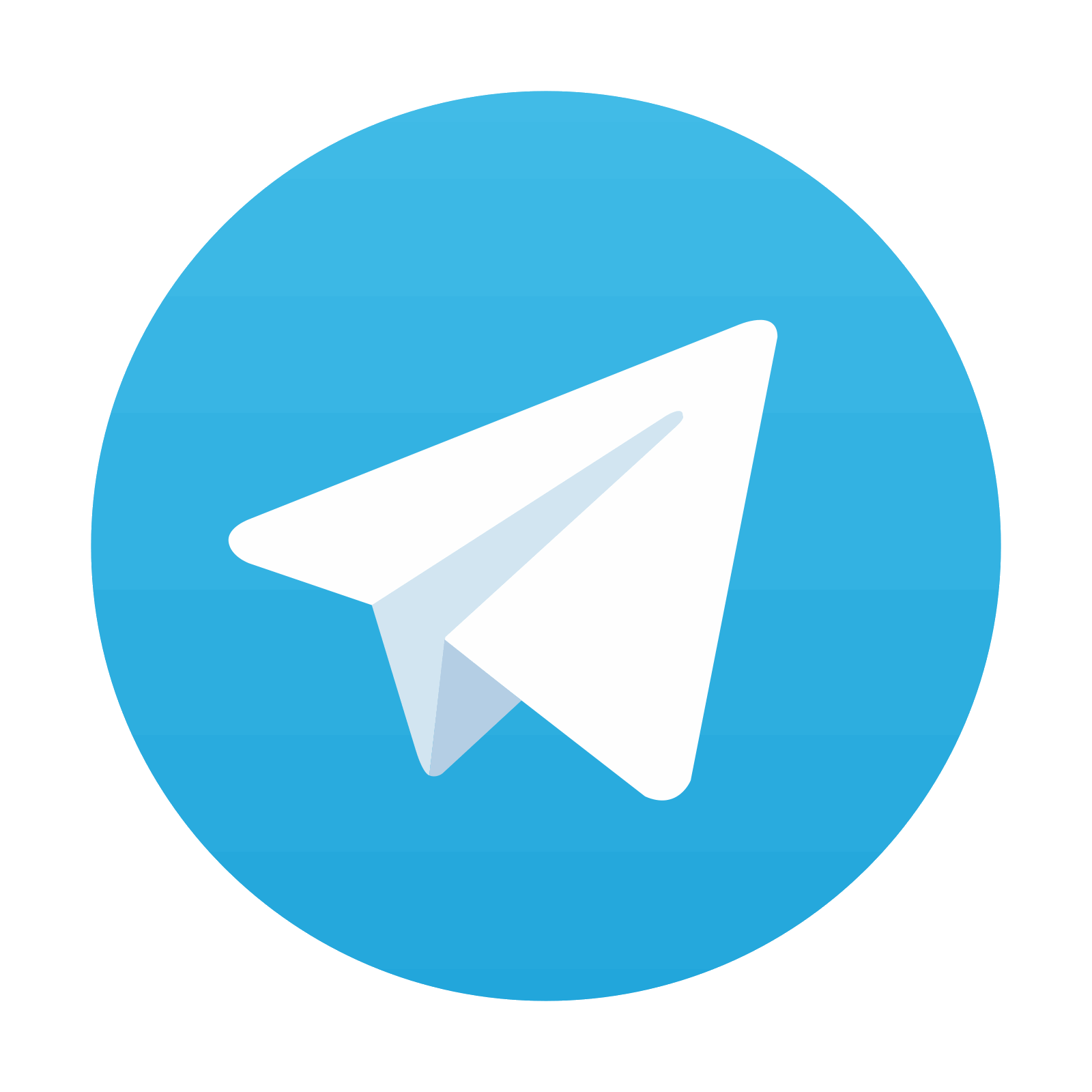
Stay updated, free articles. Join our Telegram channel
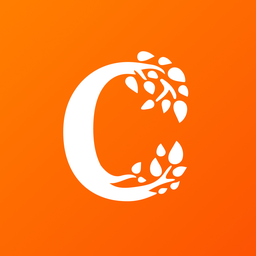
Full access? Get Clinical Tree
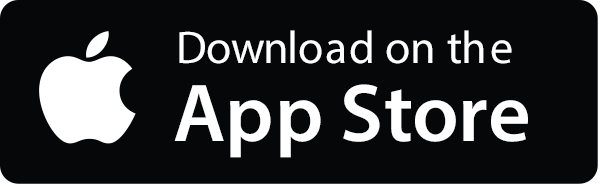
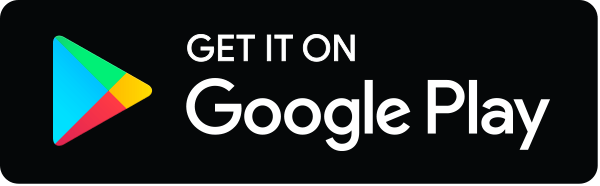