Adapted from Lim LS, Mitchell P, Seddon JM, et al. Age-related macular degeneration. Lancet. 2012;379:1728–1738.
Table 4.2 PHARMACOGENETICS AND AMD
Adapted from Chen Y, Bedell M, Zhang K. Age-related macular degeneration: genetic and environmental factors of disease. Mol Interv. 2010;10(5):271–281.
Genetic variations in genes encoding the complement proteins and regulators have been identified as protective or risk factors for AMD. Many of them are single nucleotide polymorphisms (SNPs), causing a change of a single amino acid in the polypeptide chain that could affect the binding affinity of the complement protein to its substrates. This suggests that certain individuals with some of these variations may be genetically predisposed to AMD. Variations of the complement system likely interact with environmental risk factors to determine overall risk.
Complement Factor H
Complement factor H (CFH), an important regulator of the alternative pathway, was the first complement protein to be implicated in the pathogenesis of AMD. Physiologically, CFH binds C3b and accelerates the decay of the alternative C3 convertase (C3bBb) and acts as cofactor for the inactivation of C3b by complement factor I (CFI) (37). CFH is produced locally in the eye by the RPE cells (38) and accumulates in the drusen, sub-RPE space, RPE, interphotoreceptor matrix, and choroid (38,39). Environmental factors vary CFH production by the RPE cells. In vitro studies show an increase in RPE CFH production by interferon gamma (40) and reduction in conditions of oxidative stress (38).
The CFH gene is located at chromosome position 1q23. Mutations of this gene manifest as dominant mendelian disorders (41). In 2005, three groups simultaneously (27,42,43) reported a nonsynonymous SNP in the CFH gene (rs1061170), resulting in a substitution of tyrosine by histidine at position 402 of the polypeptide (Y402H), which was important in the development of AMD. This change alters the ligand binding site of CRP (C-reactive protein), heparin, M protein, and glycosaminoglycans, probably leading to a reduced binding to cell surfaces and therefore impaired regulation of the alternative C3 convertase (44). A meta-analysis (45) suggested that this variant (Y402H) is a contributing factor in over half of all cases of AMD. Other SNPs throughout the CFH gene, including the SNP 162 V (38), resulting in substitution of isoleucine with valine residue within the C3b binding site, have been associated with AMD (32,38,41,46–50) and AMD progression (51–55).
Variations in VEGF Gene
Some authors (56–58) proposed that the SNP A/A in the allele rs3024997 and G/G re2010963 in the VEGF gene are associated with a better response to antiangiogenic (bevacizumab) therapy with regard to visual acuity outcomes. In a more recent study (59), using a multivariate data analysis and a higher number of patients, this SNP was not found to be associated with a different response to antiangiogenic therapy.
WHAT HAPPENS IN THE EYE WITH AMD?
In order to understand AMD, it is important to understand the physiologic aging of the eye. The age-related changes in the retina that predispose a person to AMD occur in the outer retina: the photoreceptors (PRs), the RPE, the Bruch’s membrane, and the choriocapillaris. Most of these changes are not clinically detectable until a late stage, when they start to affect the visual function of the patients.
The Photoreceptors
PR cells translate light into electric activity that can be understood by the brain and central nervous system. Anatomically, PR cells have four different regions: the outer segment (OS), the inner segment, the cell body, and the synaptic terminal. The OS of the PR is composed of membranous disks, which have a high concentration of visual pigment. Rhodopsin, the visual pigment of rods, is a G protein–coupled receptor that when stimulated by a photon of light undergoes a conformational change initiating a series of biochemical steps leading to the onset of the electric activity (3). Numerous studies of the human and animal retina show that excessive light exposure leads to photochemical injury causing damage to the outer segment of the PR. Excessive light exposure is considered to be an environmental factor associated with AMD (60), but the magnitude of this risk is hard to evaluate and is controversial. Even under normal light conditions, the PR incurs significant oxidative stress, due to the great energy requirement for visual phototransduction (61). This stimulation increases the oxygen-reactive species, damaging DNA and other macromolecular complexes important for the PR survival (62,63). For a long-term survival of the PR cells, it is important to have a healthy RPE participating in the visual cycle, renewing the PR OSs and producing the interphotoreceptor cell matrix (3).
The Retinal Pigment Epithelium
The RPE is a postmitotic, cuboidal monolayer of cells located between the neural retina and the Bruch’s membrane. Physiologically, it has a very high metabolic rate and performs several important functions for the retina. Of all the functions of the RPE, the most important for understanding AMD (64) are the following:
- Regeneration of bleached visual pigments (opsins)
- Formation and maintenance of the interphotoreceptor matrix and Bruch’s membrane
- Transport of fluids and ions between PRs and choriocapillaris
- Phagocytosis
The reconstitution of the visual pigment rhodopsin occurs mainly in the RPE cells, through many intermediate steps. This mechanism has a key role for normal function of both cones and rods, transforming the all-trans-retinyl esters into 11-cis-retinal (65). As a phagocytic system, the RPE is essential for the renewal of PRs (66). Each PR has hundreds of disks in its outer segment. These disks are formed by plasma membrane, containing transmembrane protein rhodopsin, which is positioned in combination with four phospholipids and docosahexanoic acid. The OS disks of the PR cells are engulfed by the RPE. In the RPE, the disks fuse with lysosomes, forming phagolysosomes, where the contents are degraded. In young, healthy individuals, most of the disks are fully degraded, and lipofuscin accumulation is minimal, but over time, the self-limited phagocytic and degradative capacity of the RPE cells becomes more and more overloaded. This incompletely degraded membrane material accumulates in the form of lipofuscin in secondary lysosomes or residual bodies (67).
Lipofuscin is a yellow-brown, autofluorescent molecule that accumulates in all postmitotic cells, particularly the RPE (68–71). The presence of lipofuscin may act as a cellular aging indicator, and its quantity in tissues may be estimated by amounts of autofluorescence present. The autofluorescence from the eye fundus, mostly derived from lipofuscin, can be clinically and noninvasively quantified, allowing for an estimate of the aging degenerative process of the eye and a diagnostic and follow-up method for patients with AMD (72). Some factors increase (vitamin E deficiency) while others decrease (oxygen-free conditions and vitamin A deficiency) lipofuscin pigment formation.
The retinoid A2E is the major fluorophore of lipofuscin (73). Once it is synthesized, it cannot be eliminated by the RPE. Precursors of A2E, all-trans-retinal and ethanolamine, are formed within the PRs (74), but the fully synthesized A2E molecule arises from the phagolysosomal compartment of the RPE cells. When it reaches a critical concentration, the metabolism of phagocytized OS lipids by the RPE is impaired. Phagocytized and oxidized OS membranes are extruded by the RPE into the Bruch’s membrane, contributing to drusen formation and membrane thickening.
A2E is toxic for the RPE. It inhibits the proton pump of lysosomes (75), causing leakage of the contents of the lysosomes into the cytoplasm of RPE cells. It inhibits phagolysosomal degradation of PR phospholipids (76) and can also damage the DNA of RPE cells. A2E also accumulates in the mitochondrial membranes, decreasing mitochondrial activity and enabling the translocation of cytochrome C and AIF (apoptosis-inducing factor) to the cytosol and nucleus, respectively. Functionally, the release of cytochrome C from the inner mitochondrial membrane generates oxidative stress and decreased electron flow, leading to impaired ATP synthesis. Both mechanisms are highly relevant for apoptosis by causing leakiness of the inner mitochondrial membrane and release of the propapoptotic proteins, activating the caspase cascade. AIF is a pro-apoptotic protein, which is strictly located in the mitochondria. Its translocation to the nucleus induces apoptosis, functionally independent from caspases (77). A2E also inhibits the normal activity of the enzyme RPE65 isomerohydrolase, a key enzyme of the visual cycle, which is responsible for the isomerization of all-trans-retinyl ester to 11-cis-retinol, the precursor of 11-cis-retinal (78). This inhibition could cause a disruption of 11-cis-retinal supply to the retina and result in PR dysfunction. A2E is known to perturb the cholesterol metabolism in the RPE cells, causing cholesterol accumulation within the late endosome/lysosome, inhibiting acid lipase and resulting in a feed-forward cycle of cholesterol accumulation in the RPE. This interferes with rhodopsin activation and PR membrane remodeling (79). A2E is also thought to be photosensitizing due to its broad light spectrum–absorbing capacities, particularly in the visible ranges, and thus generating reactive species of oxygen (80,81).
The Bruch’s Membrane
The Bruch’s membrane lies beneath the RPE and has three layers: two collagenous layers on the top and bottom and a central elastic layer (82). Proteoglycans are an important constituent of the Bruch’s membrane (83). Their negative charges hinder the passage of the positively charged macromolecules necessary for RPE maintenance. Changes in the Bruch’s membrane begin prior to the development of clinically significant AMD. As soon as the third decade of life, basal lamellar deposits and membranous debris, precursors of AMD, begin to appear (84,85).
Hard drusen appear between the basement membrane of the RPE and the inner collagenous layer of the Bruch’s membrane in the third decade of life (86). It is not well understood how drusen develop. Drusen have a complex structure. They have a core of glycoproteins and an outer dome containing crystallins, chaperones, apolipoprotein E, vitronectin, inflammation-related proteins (as amyloid P, C5, C5b-9 complement complex), and fragments of RPE cells (87–91). Basal laminar deposits (BLD) contain granular electron-dense material, coated membrane bodies, and wide- or long-spaced fibrous collagen (92–96).
Vesicles and membranous debris, referred to as basal linear deposits, accumulate beneath the RPE basement membrane and correlate histopathologically to soft drusen (93,97).
Features of the normal aging of the Bruch’s membrane include increased lipid concentration (99–104), calcification (98), and decreased concentration of metalloproteinases. The most common lipid in the aged Bruch’s membrane deposits is the phospholipids, derived from local cells rather than blood (101). There is an age-related decrease in adhesion molecules, laminin and fibronectin, that is inversely correlated with the lipid content of the Bruch’s membrane (100). In contrast, another study concluded that the main lipid component of these deposits was esterified cholesterol, similar to depositions in other membranes throughout the body that occur with age, suggesting a blood origin of these rather than cellular (105).
These changes contribute to the thickening of the Bruch’s membrane, interfering in normal function of this membrane by decreasing the hydraulic conductivity. Thus, fluids pumped out by the RPE cells, toward the choroid, encounter the resistance provided by the thickened membrane, leading to the formation of serous RPE detachments. Such morphologic changes lead to alterations in cellular adhesion, which could cause the triggering of an apoptosis (106,107).
Choriocapillaris
The choriocapillaris is the vascular layer of the eye. Between the retina and the sclera, it is formed by an extensive fenestrated vascular network derived from the perforating ciliary arteries, branches of the ophthalmic artery. Its main role is to provide oxygen and macromolecules to the outer retina. Over the years, the choriocapillaris becomes less fenestrated and the lumina are reduced, causing less delivery of oxygen and macromolecules to the retina (98,108,109). This change results in hypoxia and activation in the transcription of genes such as erythropoietin (for PR protection) (110) and vascular endothelial growth factor A (VEGF-A) (111,112), causing the development of CNVM.
Choroidal Neovascular Membranes
CNVMs are fibrovascular complexes that arise from the choroid, penetrating the Bruch’s membrane and proliferating in the sub-RPE and subretinal space. This complex is formed by two major components: neovascular sprouts and fibroblasts. Proliferation of this fibrovascular complex leads to disturbances in the normal architecture of the choriocapillaris, Bruch’s membrane, RPE, photoreceptors, and retina.
Since the Macular Photocoagulation Study in the 1970s, CNVM was classified based on its localization relative to the fovea (extrafoveal, juxtafoveal, and parafoveal) and the pattern of dye leakage on fluorescein angiography (FA) (classic and occult). Advances in diagnostic techniques for CNVM, particularly indocyanine green angiography (ICGA) and optical coherence tomography (OCT), allowed determination of the retinal layer in which CNVMs are located.
A new classification for CNVM based on FA, OCT, and ICGA patterns includes type 1 (CNVM beneath RPE), type 2 (subretinal CNV), and type 3 (retinal angiomatous proliferation) (113).
UNDERSTANDING HOW ALL THESE CHANGES LEAD TO AMD
The aging process of the eye affects the PRs, the RPE, the Bruch’s membrane, and the choriocapillaris. In these tissues, accumulation of substances, functional changes, and apoptosis may occur, and important pathophysiologic mechanisms are activated by these changes.
Angiogenesis
Angiogenesis, leading to the formation of CNVM, is one of the most important pathophysiologic features of late AMD. In angiogenesis, blood vessels grow in adult tissue from sprouts on preexisting vessels. This process involves several steps: cell migration, proliferation, and survival; vascular maturation; wall remodeling; and degradation of the extracellular matrix (114–116). Angiogenesis has a complex molecular regulation, representing a balance between the angiogenic and the antiangiogenic factors, affected by different pathologic states such as hypoxia, ischemia, or inflammation (2).
VEGF-A, Hypoxia, Inflammation, and Oxidative Stress
VEGF-A is a remarkable angiogenic factor. This homodimeric glycoprotein with a molecular weight of 45 kDa (117) has four major isoforms, distinguished by their molecular weight, acidity, and whether they are bounded to heparin.
VEGF-A is synthesized by RPE cells constitutively and is elevated in early forms of AMD and hypoxia. VEGF-A is highly selective for endothelial cells. It has diffusion characteristics that allow it to reach its target. It has several regulation mechanisms and affects the angiogenesis mechanism in different stages, including endothelial cell proliferation, survival, and migration, and also promotes the vascular hyperpermeability (118). VEGF receptors, VEGFR-1 (Flt-1), VEGFR-2 (Flk-1/KDR), and VEGFR-3 (Flt-4), are expressed predominantly in endothelial cells and to a lesser extent on monocytes and macrophages (119). Normal human choriocapillaris expresses all VEGF receptors in the choriocapillaris endothelium next to the RPE layer, suggesting a paracrine relation between RPE cells and the choriocapillaris (120). VEGF has also been shown to have survival function in the retina (121) and has a role in the maintenance of the adult choriocapillaris via stimulating the formation of fenestrations (122).
Hypoxia
The role of hypoxia on the pathogenesis of AMD is controversial. While some studies (123) showed that patients with AMD have decreased blood flow in the choriocapillaris, others (124) could not confirm a decrease in blood flow but found a decrease in pulse amplitude. It also has been theorized that the previously described age-related changes in the Bruch’s membrane may limit the diffusion of oxygen and create an ischemic environment. Physiologically, pO2 decreases linearly with distance from the choriocapillaris to the inner segment of the PRs. Under normal conditions, little of the O2 in the choriocapillaris blood is extracted. Some studies have shown that as perfusion decreases, the oxygen extraction from the choriocapillaris increases (125), showing that there is significant reserve. This suggests that hypoxia is not the most important factor in causing an imbalance between angiogenic and antiangiogenic factors.
Inflammation
Studies of excised choroidal membranes and the growth patterns of CNVM suggest more is involved than just ischemia in driving neovascularization. Histologic studies of the CNVM show vascular endothelium as well as a variety of inflammatory cells, such as lymphocytes, macrophages, and foreign body giant cells (126,127), similar to granulation tissue on a wound-repair response (128). Activated macrophages secrete proteolytic enzymes, collagenase, and elastase, which can erode an attenuated Bruch’s membrane and thereby assist the migration of choroidal capillaries (129,130). Also, inflammatory cells produce cytokines such as interleukin-1, which induce VEGF, as shown in cultured choroidal fibroblasts (131). In one study (132), the amount of VEGF was found to be proportional to the number of macrophages in the specimen, suggesting that inflammation is important for the regulation of angiogenesis. Therefore, upregulation of VEGF may be the link between inflammation and promotion of new blood vessels in an eye with AMD.
Before the formation of the CNVM, there are signs of inflammation in an eye with AMD. Drusen deposits between the Bruch’s membrane and RPE may contain bioactive fragments of complements (C3a and C5a) (133). These substances induce VEGF expression and have significant chemotactic activity that further invites inflammatory cells to the macular region (134). Another key actor of the complement system in AMD is the CFH. CFH binds and inactivates C3b deposited on intact host cells, allowing destruction of foreign or damaged cells (135). This is achieved through an important locus, domain 7 on the molecule (136–139). Domain 7 binds to heparin or sialic acid on host cells. Therefore, it is possible that alterations in domain 7 or the heparin/or sialic acid binding region of factor H could lead to or augment the destruction of normal or injured ocular cells as well as other foreign cells in an appropriate environment (44).
Extracellular drusen also contain advanced glycation end products (AGE), high levels of oxidized low-density lipoproteins (ox-LDL), and oxysterols (87,140,141). The receptor for AGE (RAGE), normally not markedly expressed in the retina, although highly accumulated in RPE cells, PRs, and choriocapillaris in advanced AMD (142,143), activates the nuclear factor kappa B (NFκB), master regulator of both innate and adaptive immunity. NFκB is a central regulator of IL-6, a cytokine that has been shown to be an important regulator of choroidal neovascularization (144–146), and VEGF. Damaged RPE and oxidized proteins and lipids in the Bruch’s membrane have been postulated to activate and recruit dendritic cells from the choroid, enhancing the immune response in this area (88,147).
In a laser model of CNV, CD18 and ICAM-1 are expressed during development of CNV. Targeted disruption of either of these inhibits the development of CNVM. Animals models of CNV have been developed that mimic many aspects of CNVM in AMD. Mice expressing monocyte chemoattractant protein-1 (MCP-1) or its cognate, CC chemokine receptor-2, developed drusen, lipofuscin accumulation, geographic atrophy, and CNV (148). Depletion of the monocyte cell lines inhibits experimental CNV (149–151).
With time, the neovascularization appears to “burn out,” leaving a cicatricial mass almost completely devoid of vessels. If ischemia is the only cause of neovascularization, one would expect these vessels to regress, which would increase the amount of ischemia present.
The Complement System
The complement system plays a fundamental role in host defense against pathogens, elimination of immune complexes and apoptotic cells, and adaptive immune responses. It consists of over 40 proteins and regulators found in the systemic circulation and some tissues. Three different activation pathways exist, each one with different triggers: the classical pathway (triggered by an antibody–antigen complex), the alternative pathway (triggered by binding to a host cell pathogen surface), and the lectin pathway (triggered by polysaccharides on microbial surfaces). These three pathways converge on the terminal complement complex (TCC), a multiprotein complex, formed from the binding of C5b to the plasma complement proteins C6, C7, C8, and C9 (152).
Components of the classic, alternative complement pathways and TCC have been found to be present in drusen (153), especially in older eyes with AMD.
Alterations of the complement system or its regulators can lead to damage of the tissues and is known to be implicated in a wide spectrum of diseases. Several studies suggest that complement plays a key role in the development of AMD (154).
Other Growth Factors Involved in Angiogenesis
Several other growth factors are involved in the regulation of angiogenesis in the eye. These mechanisms help us to understand why conventional therapies do not succeed in some cases and spur the development of new therapeutic strategies.
- Pigment epithelium–derived factor (PEDF), produced by the RPE, is a neurotrophic PR growth factor and generally is thought to inhibit angiogenesis. It is the main antiangiogenic cytokine in the eye. Under hypoxic conditions and wet AMD, secretion of PEDF is decreased, thereby allowing the endothelial mitogenic activity of VEGF to go unchecked (154–156). Some paradoxical effects have been identified (155,157).
- Platelet-derived growth factor (PDGF) is involved in the development and differentiation of vessel walls. It is required to recruit pericytes and to promote maturation of the microvasculature. It is a potent chemoattractant, dedifferentiater, and mitogen for both fibroblasts and RPE cells (158–160). The potential therapeutic effect of anti-PDGF therapy still needs to be studied (161).
- Basic fibroblast growth factor (bFGF) is a potent angiogenic molecule in vivo and is produced by a variety of cell types including vascular endothelial cells of the choriocapillaris, fibroblasts, astrocytes, and RPE cells (162,163). bFGF induces the secretion of VEGF and HGF by Müller glial cells and stimulates cell proliferation (163–165). One study comparing the antiangiogenic effect of bevacizumab (anti-VEGF) alone and combined with anti-bFGF antibodies observed that targeting bFGF in addition to VEGF could show synergistic effects in CNV treatment (166).
- Angiopoietin-2, detected in CNVM lesions (167), is upregulated by hypoxia and VEGF. It may facilitate hypoxia and VEGF-induced neovascularization by destabilizing existing vasculature (168,169).
- Metalloproteinases are enzymes that remodel the extracellular matrix in neovascular processes. They can be found in AMD CNVM lesions, and they are upregulated by the VEGF (170,171).
Oxidative Stress
Oxidative stress refers to a progressive cellular damage caused by reactive oxygen species (ROS) contributing to protein misfolding and evoking functional abnormalities during RPE cellular senescence (172). Light can induce the formation of reactive species, which in turn can lead to the formation of dysfunctional or toxic molecules of lipids and proteins or damage the DNA. Oxidized molecules are formed in the PR OSs as a normal part of daily life. Over the years, the amount of these molecules increases because the mechanisms needed to clear the reactive species out of the cells become insufficient (173). Oxidative stress also interferes with the capacity of the heat shock proteins and the ubiquitin–proteasome way to repair or degrade damaged proteins (174–176).
Aging primarily influences the oxidative balance between oxidizing factors and antioxidants. Other factors, such as cigarette smoking and alcohol consumption, aggravate oxidative imbalance and DNA damage (177). Antioxidant genes (178) and variants of the CFH gene influence the role of oxidative stress in AMD (179).
A wide variety of oxidative lesions to DNA have been described, including single- and double-stranded DNA breaks and the development of cross-linked lesions (180,181). The cell responds to genomic damage through repair processes employing a large number of proteins. The cell may turn off growth and replication until the repair process is complete (182). Responding to the Samurai law of biology (better dead than wrong), those cells unable to repair their DNA damage undergo apoptosis.
To help protect against inappropriate oxidation, there are basically three levels of protection: molecular, cellular, and tissue. On the molecular level, the cell has antioxidant vitamins and enzymes. These include vitamins C and E, superoxide dismutase, catalase, glutathione transferase (183), glutathione reductase, and glutathione peroxidase. The antioxidants may limit inappropriate oxidation in the first place or may terminate propagation reactions. On a cellular level, two main responses may occur. The cell may try to adapt to the oxidative stress by increased activation of transcription factors and proteins (184) that helps control gene expression of antioxidant enzymes. The cell could also start the apoptosis mechanism. Tissue mechanisms for responding or protecting against oxidative stress include complex intercellular signaling pathways as discussed below.
Scavenger receptors (185,186), in particular CD36, are present on the RPE cell and participate in phagocytosis of spent OSs. It is possible that ordinary everyday exposure of CD36 receptor to oxidized lipids in the PR OS helps maintain the constitutive secretion of VEGF by RPE cells. Excessive secretion of VEGF by RPE cells is supposed to be one factor responsible for the initiation of CNV. This raises the possibility that excessive exposure to oxidative damage may lead the RPE cells to secrete excessive VEGF. Armstrong et al. (187) have shown that injection of lipid peroxide derivate into the vitreous cavity caused retinal neovascularization that persisted for 4 weeks. After the injection, there was a cascade of cytokines secreted, including VEGF. The Bruch’s membrane has an exponential increase in lipids with age. The lipids preferentially accumulate in the same region where the neovascularization grows. The Bruch’s membrane has no known intrinsic mechanism to protect against oxidative damage to lipids, which accumulate over time. This mechanism could be important in the formation of CNVM in AMD.
Lipid peroxides not only accumulate in the Bruch’s membranes but also accumulate in atherosclerotic plaques. In atherosclerotic vessels, the body mounts an aggressive cell-mediated mechanism to contain the oxidized material (188,189), principally using vascular endothelial cells and macrophages also stimulating the production of VEGF by these cells. The body has a number of defined strategies and methods for dealing with degenerating cells and tissue. Many of the same strategies and methods that are used in atherosclerosis of vessel walls are also used in the eye. Injection of these lipids has led to ocular neovascularization in the rabbit (190–192).
As discussed, the accumulation of oxidative damage may be a cause of neovascularization, but the same oxidative damage may also induce a senescent aging phenotype (193), with possible apoptosis. Oxidative damage can lead to an increased accumulation of lipofuscin within RPE cells, a finding linked to the development of geographic atrophy.
CONCLUSION
In this chapter, we discussed the etiology of age-related macular disease. Epidemiologic and genetic factors, clinical classification, anatomical changes, and how these changes affected the development and evolution of the AMD were discussed. While a complete understanding of AMD and its pathophysiology is still lacking, new evidence and understanding are rapidly accumulating. There is not a single gene defect or a single molecular disturbance that causes AMD. It is a complex, multifactorial disease that may require a variety of treatment strategies to preserve vision for our patients.
REFERENCES
1.Klein R, Chou CF, Klein B, et al. Prevalence of age-related macular degeneration in the US Population. Arch Ophthalmol. 2011;129(1):75–80.
2.Bressler SB. Introduction: understanding the role of angiogenesis and antiangiogenic agents in age-related macular degeneration. Ophthalmology. 2009;116:S1–S7.
3.American Academy of Ophthalmology. Retina and Vitreous. Basic and Clinical Science Course Section 12. 2011–2012.
4.Age-Related Eye Disease Study Research Group. Risk factors for the incidence of advanced age-related macular degeneration in the Age-Related Eye Disease Study (AREDS) AREDS Report No. 19. Ophthalmology. 2005;112(4):533–539.
5.Klein R. Epidemiology. In: Berger JW, Fine SL, Maguire MG, eds. Age-related macular degeneration. St. Louis, MO: Mosby; 1999:31–55.
6.Seddon J. Epidemiology of age-related macular degeneration. In: Schachat AR, ed. Medical retina, vol 2, 3rd Ed. St. Louis, MO: Mosby; 2001:1039–1050.
7.Smith W, Assink J, Klein R, et al. Risk factors for age-related macular degeneration: pooled findings from three continents. Ophthalmology. 2001;108:697–704.
8.Hyman LG, Lilienfeld AM, Ferris FL III, et al. Senile macular degeneration: a case-control study. Am J Epidemiol. 1983;118:213–227.
9.Christen WG, Glynn RJ, Ajani UA, et al. Age-related maculopathy in a randomized trial of low-dose aspirin among US physicians. Arch Ophthalmol. 2001;119:1143–1149.
10.Klein R, Klein BE, Moss SE. Diabetes, hyperglycemia, and age-related maculopathy. The Beaver Dam Eye Study. Ophthalmology. 1992;82:1527–1534.
11.Smith W, Mitchell P, Wang JJ. Gender, oestrogen, hormone replacement and age-related macular degeneration: results from the Blue Mountains Eye Study. Aust N Z J Ophthalmol. 1997;25(Suppl):S13–S15.
12.Smith W, Mitchell P, Leeder SR, et al. Plasma fibrinogen levels, other cardiovascular risk factors, and age-related maculopathy: the Blue Mountains Eye Study. Arch Ophthalmol. 1998;116:583–587.
13.Tomany SC, Wang JJ, van Leeuwen R, et al. Risk factors for incident age-related macular degeneration. Pooled findings from 3 continents. Ophthalmology. 2004;111:1280–1287.
14.Snow KK, Cote J, Yang W, et al. Association between reproductive and hormonal factors and age- related maculopathy in postmenopausal women. Am J Ophthalmol. 2002;134:842–848.
15.Edwards DR, Gallins P, et al. Inverse association of female hormone replacement therapy with age-related macular degeneration and interactions with ARMS2 polymorphisms. Invest Ophthalmol Vis Sci. 2010;51(4):1873–1879.
16.Chumbley LC. Impressions of eye diseases among Rhodesian Blacks in Mashonaland. S Afr Med J 1977;52:316–318.
17.Gregor Z, Joffe L. Senile macular changes in the black African. Br J Ophthalmol. 1978;62:547–550.
18.Cho E, Hung S, Willet WC, et al. Prospective study of dietary fat and the risk of age-related macular degeneration. Am J Clin Nutr. 2001;73:209–218.
19.Parekh N, Voland RP, et al; CAREDS Research Study Group
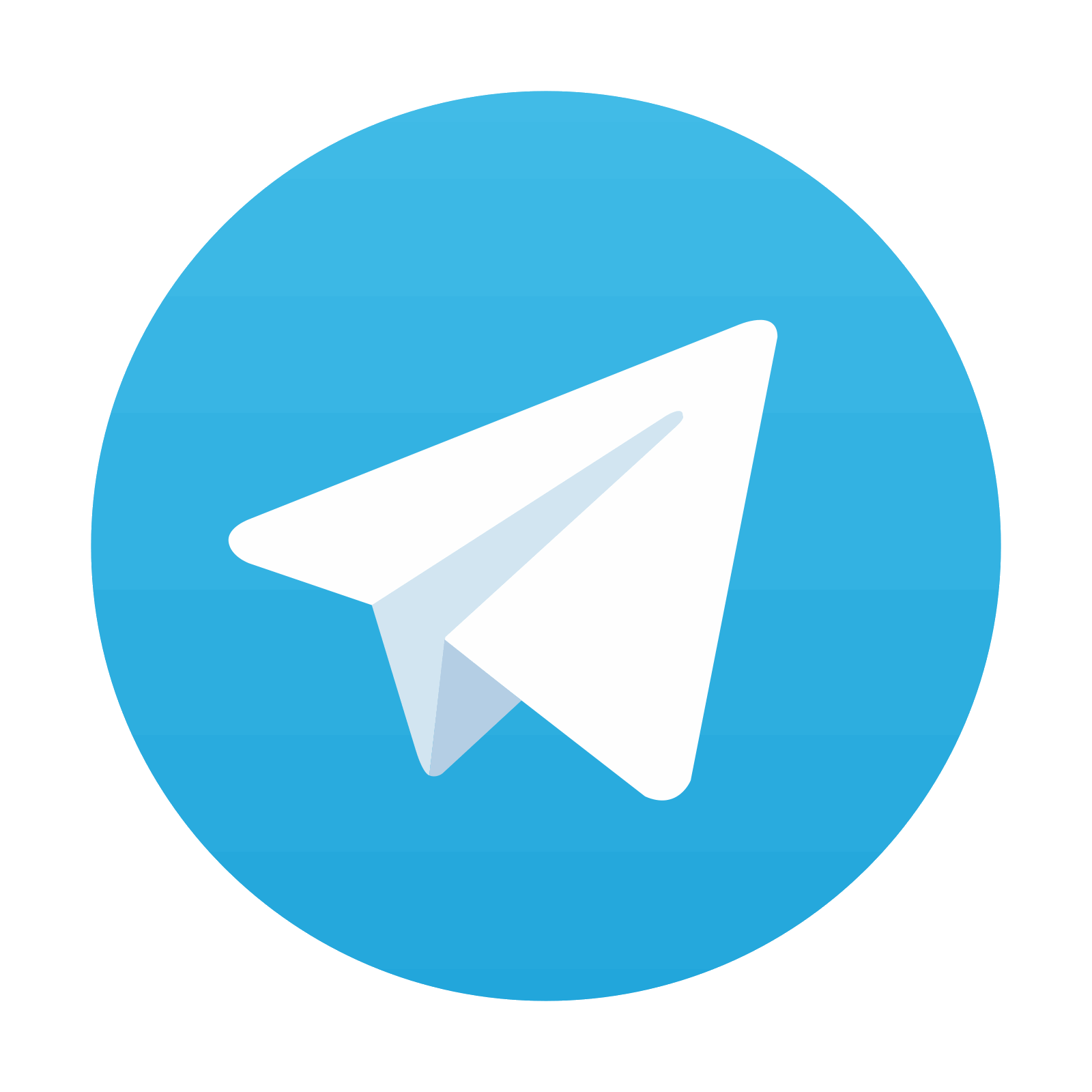
Stay updated, free articles. Join our Telegram channel
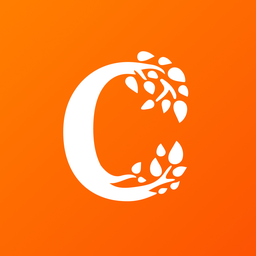
Full access? Get Clinical Tree
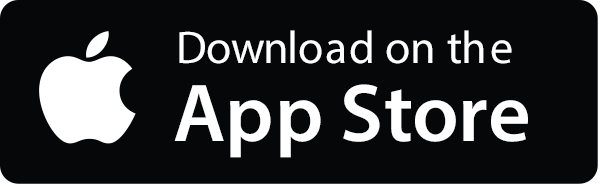
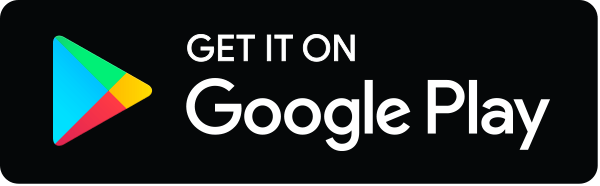