At present, there are two general approaches by which therapeutic AAV-mediated gene transfer might be useful in the context of ocular disease. First, AAV-mediated gene therapy has the potential to correct the specific gene defect in conditions where the defect is well understood. Correction of an ocular genetic defect requires gene delivery to the defective cells and has been successfully used to slow photoreceptor loss in several rodent models of primary photoreceptor diseases. Nevertheless, there are many ocular conditions where no specific genetic defect has been characterized. It is likely that many of these diseases will turn out to involve pathology more complex than a well-characterized mutation in a single gene. In such circumstances, a second strategy for gene therapy may be useful. This involves using gene transfer to reduce loss of function by ameliorating the effect of the primary defect. For instance, AAV-mediated transfection of retinal cells with the gene for basic fibroblast growth factor-2 (FGF-2), glial cell line–derived neurotrophic factor, and ciliary neurotrophic factor have been demonstrated to slow photoreceptor loss in rat models of retinitis pigmentosa (37).
Transduction of RPE cells and photoreceptors is most efficiently achieved by subretinal injection of AAV. The subretinal space has a relatively high degree of immune privilege, and typically, very little evidence of inflammation is seen in the vicinity of the injection site. Subretinal injection induces a bleb of concentrated virus in intimate contact with photoreceptors and RPE cells. On the other hand, intravitreal injection can be used to transfect Müller cells and retina ganglions cells (38). The barrier and binding qualities of the internal limiting barrier, however, appear to limit easy access to the subretinal space, from the vitreous, for rAAV. In theory, the potential for a host immune response to AAV is greater following intravitreal injection, and the possibility exists of an AAV-specific systematic antibody response.
Several developmental processes, including mitosis, migration, differentiation, cell death, and synaptic formation, may affect the cellular uptake of the virus vector, the distribution of the particles, and the persistence of transgene expression. On the other hand, the time of virus administration profoundly affects the tropism, efficiency, and distribution of the transgene (14).
One of the major limitations of AAV as a useful therapeutic vector is the relatively small amount of passenger DNA that can be incorporated. Although genes up to 6.0 kb have been packaged into AAV, these overstuffed viruses were not active. The usual packaging limit for AAV appears to be 5.1 to 5.3 kb. Nevertheless, by exploiting the intermolecular rearrangement, it is possible to overcome this problem. By way of example, genes larger than 4.7 kb can be split between two different AAV vectors.
Beltran et al. (39) reported the use of AAV-2/5–vectored human RPGR with human IRBP or GRK1 promoters in a canine model of X-linked retinitis pigmentosa cased by a GTPase regulator (RPGR) gene, which encodes a photoreceptor ciliary protein. They were able to show with in vivo imaging, preserved photoreceptor nuclei, and inner/outer segments that were limited to treated areas. Both rod and cone photoreceptor function were greater in treated (three of four) than in control eyes. Histopathology indicated normal photoreceptor structure and reversal of opsin mislocalization in treated areas expressing human RPGR protein in rods and cones. Postreceptoral remodeling was also corrected: there was reversal of bipolar cell dendrite retraction evident with bipolar cell markers and preservation of outer plexiform layer thickness. At this time, no clinical trials have begun.
Herpes Simplex Virus Vectors
The herpes simplex virus (HSV) is a DNA virus that has natural tropism for neurons. Thus, it may be useful in applying gene therapy to diseases of the nervous system, including the retina. HSV can infect nonneuronal tissue as well and does not require cell division to integrate into the host genome. Gene transfer using HSV utilizes either replication-defective mutants or multiple deletion mutants that have a limited capacity for replication, but they may be able to deliver genes locally within tissue with a limited infection. The ability of HSV to exist in a latent state likely makes this vector efficacious in producing stable transgene expression within host neurons. Moreover, the HSV viral genome is large and has the capacity to carry more than 30 kb of foreign DNA, significantly more than other vectors.
The HSV thymidine kinase gene (HSV-tk) has been used extensively in suicide gene therapy studies. The viral thymidine kinase has a markedly higher affinity for the prodrug ganciclovir than does the cellular thymidine kinase. Ganciclovir is converted to a cytotoxic metabolite with significantly higher efficiency by HSV-tk–transduced cells, and thus preferentially kills the transduced cell (31).
RNA Virus Vectors
Retroviruses are a family of RNA viruses that can infect cells and integrate into the genome of the host cell. RNA viruses are surrounded by a lipid envelope. Upon entry into the target cell, the RNA is released and reverse transcribed into double-stranded DNA that is stably integrated into the host DNA. Transgenes delivered by retroviruses demonstrate long-term stable gene expression and may be vertically transferred to daughter cells. Most retroviral vectors cannot enter the nucleus directly; they require cell division to infect the host cell following breakdown of the nuclear envelope.
To date, retroviral vectors are the most frequently employed platform in human clinical trials. The retrovirus vector transduces primarily proliferating cells of rodent and human origin that express the cellular receptor Ram-1 (40,41). They have been used as effective gene delivery tools in cells from the liver, retina, skeletal muscle, and the central nervous system. Retrovirus does not, however, transduce nondividing cells with an efficiency that translates into clinical utility at this time. Therefore, most retroviral vectors have limited application for targeting neural retinal cells or other ocular cells, which are largely postmitotic, in the postnatal period.
Lentiviruses are a subclass of retroviruses that includes the HIV family. Like all retroviruses, lentiviruses integrate a DNA copy into the host genome, but they encode proteins that allow them to form a complex with the nuclear envelope and to transit the pores of an intact nuclear membrane. Thus, lentiviruses can infect dividing and nondividing cells.
The ability of lentiviruses to integrate into nondividing cells relies on nuclear localization signals present in the preintegration complex that allow its entry into the nucleus without the need for nuclear membrane fragmentation. Transgenes integrate into the host genome, but viral genes do not; thus, there is less risk of generating recombinant retrovirus. Despite modifications to render the virus replication incompetent, there is appropriate concern about the clinical use of such vectors because of the theoretical possibility of generating a wild-type HIV virus during production or from viral recombination in the host (31). Bovine immunodeficiency virus (BIV) is a lentivirus that is not known to cause human disease. Subretinal injection of a BIV.GFP vector has been shown to produce a quick and prolonged transduction of RPE cells without an evident inflammatory response (42).
Table 24.1 summarizes the characteristics of gene transfer with viral vectors.
Figure 24.1 Intravitreous injection in the mouse eye.
VIRAL VECTOR DELIVERY
Intravenous Vector Delivery
At the time of this writing, intravenous delivery of gene therapy vectors remains synonymous with systemic gene therapy. It is conceivable that advances in vector targeting, development of tissue-specific promoters, and further narrowing of vector tropism will lead to intravenous delivery of vectors that are highly specific for distant cell types, in the future. At this time, however, intravenous delivery is generally attended by systemic distribution of vector, transgene, and the products of transgene expression. The potential for both local and distant therapeutic effect as well as toxicity must be considered. Intravenous injection of adenoviral vectors expressing a transgene sequence coding for endostatin has been reported to inhibit systemic angiogenesis and tumor growth (43) as well as choroidal neovascularization (CNV) in a mouse model (8). Intravenous delivery of viral vector in the mouse has been previously described (8,43). Intravenous delivery of vector exposes the vascular endothelial cells of the retinal and choroidal vessels directly to the vector.
Intravitreous Vector Delivery
Intravitreous delivery of viral vectors exposes the cells that line the interior of the eye to vector. In mice, intravitreous injection is carried out on anesthetized animals under a dissecting microscope. The procedure has been described in detail elsewhere (5) (Fig. 24.1).
Following intravitreous delivery, viral vectors have direct access to the vitreous cavity and the cells that line the posterior and anterior ocular cavity.
In rats and larger animals, intravitreous delivery can be carried out in a manner analogous to intravitreous delivery in humans. Intravitreal injection is much more convenient in humans than it is in small animals. It is done routinely in the clinic, with only rare complications, chiefly, endophthalmitis and retinal detachment.
Subretinal Vector Delivery
Subretinal injection exposes both the photoreceptors and RPE directly to the vector. Various approaches to carrying out subretinal injection in mice have been described (4,8,44,45). Success has been achieved with a transscleral and transchoroidal approach (4,44,45), and with a transscleral and transvitreal approach as described by Mori et al. (8). The transscleral and transvitreal approach requires direct visualization of the retina and has been described elsewhere and is shown in Figure 24.2.
Figure 24.2 Subretinal injection in the mouse eye. Following subretinal delivery, viral vectors have direct access to the subretinal space including photoreceptor outer segments and the RPE.
Periocular Injection of Vectors
Periocular gene transfer provides an alternative to intraocular vector delivery when the vectored transgene results in a secreted protein and the protein has properties that allow it to diffuse into the eye. In prior work, Gehlbach et al. (9) have shown that periocular vector delivery results in levels of pigment epithelium–derived growth factor or the secreted extracellular domain of vascular endothelial growth factor (VEGF) receptor 1 that are significantly elevated in the choroid and sufficient to inhibit choroidal neovascular membrane formation in mice. Periocular injection in mice has been described elsewhere and is depicted in Figure 24.3.
Figure 24.3 Periocular injection in the mouse eye. Following periocular delivery, viral vectors have direct access to the subconjunctival space including episclera and conjunctiva. Access to orbital tissues including muscle, connective tissue, and lacrimal gland is also present.
Ribozyme Therapy
Ribozymes are RNA-based enzymes that can cleave specific mRNA sequences. Mutation-specific cleavage of the transcript functionally silences the mutant allele by preventing synthesis of the abnormal protein from the transcript. The strategy used is to construct ribozymes that identify unique mutations or that permit binding to targeted, accessible sites in the mRNA transcript. Ribozyme therapy is effective in delaying retinal degeneration in animal models of dominant retinal degeneration, even after the degenerative process has begun.
Growth Factor Gene Therapy
Gene therapy using trophic growth factors is another approach to enhancing the survival of retinal photoreceptors in dominant retinal generation. For instance, the intravitreal injection of basic fibroblast growth factor (bFGF) delays retinal degeneration in some animal models (46).
Successful treatment with growth factors may also be achieved by genetically modifying cells ex vivo and then implanting them within the eye to act as a reservoir of trophic factors that bathe the retina by slow release into the vitreous.
Apoptosis has been implicated in retinal development and degeneration, but the specific apoptotic pathways are incompletely understood. Apoptosis is a process of programmed cell death without an ensuing inflammatory response. This neat packaging of cellular components allows for the precise removal of tissue during developmental remodeling, particularly in the retina. The retina develops from a single layer of undifferentiated ventricular neuroectoderm to a mature retina containing three cell layers of postmitotic, fully differentiated cells: the ganglion cell layer, the inner nuclear layer, and the outer nuclear layer. Extraneous cells unable to make functional neural connections are eliminated by apoptosis. Beyond this involvement in ocular development, apoptosis rarely occurs in a normal, healthy retina but has been implicated in both inherited and acquired retinal degenerations. The molecular pathogenesis of these retinal degenerations is still unclear, but apoptosis is the final common pathway in many retinal diseases, ranging from glaucoma to AMD, retinitis pigmentosa, and retinal detachment (47). Several proapoptotic and antiapoptotic genes have been identified. The use of growth factor gene therapy could help in rescue and/or prevent the apoptotic phenomenon in the target cells.
Antisense Gene Therapy
Antisense gene therapy is based on the use of synthetic, short DNA sequences that are designed to be complementary to a targeted mRNA molecule. This short DNA sequence is capable of forming a stable DNA–mRNA heteroduplex that prevents the translation of the associated protein from the transcript.
GENE THERAPY IN CLINICAL USE
Several retinal diseases have been proposed as targets for gene therapy. Retinal degenerations are particularly appealing since many have little or no conventional therapy, poor visual prognosis, and well-established genetic causes. In 2012, Jacobson et al. (48) reported on the 3-year results of a phase I/II clinical trial of the rAAV2-RPE65 vector injected subretinally for the treatment of LCA caused by RPE65 mutations. The study included 15 eyes from 15 patients 11 to 30 years old. The patients were broken into five cohorts that represented four dose levels and two different injection strategies. No systemic toxicity was reported, and ocular toxicity was related to surgical implantation. Visual improvement was seen in all patients but was modest. Improvement was also seen in the contralateral, control eyes, but this was statistically less than that of the experimental eyes. The study concluded that extrafoveal implantation is safer for these patients than foveal treatment. Additional studies are in progress to quantify the treatment benefit and long-term side effects.
GENE THERAPY AND AGE-RELATED MACULAR DEGENERATION
In wet AMD, a destabilization of the retinal and choroidal microenvironments leads to the formation of new blood vessels, which ultimately results in a decrease of visual acuity. Degenerative changes of the RPE and Bruch’s membrane are the primary factors responsible for the disease. The pathophysiology of the disease is still incompletely understood. The putative role of specific genes in the degenerative process in AMD is less clear. Although certain genes may predispose some patients to develop AMD, the genetic linkage remains controversial and, to date, the genetics of AMD are emerging. Genetic susceptibility to AMD is probably multifactorial and thus will probably not be amenable to single gene therapy directed at the germinal line. In the absence of a well-defined genetic defect that gives rise to AMD, gene therapy will likely focus on somatic therapy using growth factors and antiapoptosis therapy to prolong the survival of the RPE and retinal photoreceptors, and these studies supported by the research community and commercial interests are under way.
Growth Factor Gene Therapy for AMD
There is experimental evidence that growth factors play an important role in maintaining the health of RPE cells and in enabling them to respond to injury. RPE cells express growth factors, and their receptors demonstrate the autocrine and paracrine functions of these substance. Theoretically, it may be possible to enhance RPE cell survival by somatic modulation of growth factor gene expression in patients with AMD. For instance, age-related phagocytic dysfunction and incomplete digestion of photoreceptor membranes by the RPE result in loss of RPE cells and in geographic atrophy, perhaps due in part, to the cytotoxicity of these deposits on the surrounding cells. Enhancing phagocytic activity in aging RPE cells using gene therapy is one potential approach to the treatment of AMD. bFGF has been shown to stimulate phagocytic activity and prolong retinal survival in animal models (31). An important number of other growth factors and secondary messengers of the intracellular signaling pathways have demonstrated protective effects on the retinal neurons in animal models of retinal degeneration. Gene transfer and expression of these growth factor proteins may similarly inhibit retinal degeneration by a neuroprotective effect in AMD (49).
The RPE synthesizes proteins that are antiangiogenic, such as tissue inhibitors of metalloproteinases and PEDF. Thus, potential gene therapy applications to CNV include antiangiogenic growth factor gene therapy, antisense or ribozyme therapy directed at angiogenic factors, and suicide gene therapy directed at neovascular tissue. Moreover, it was demonstrated that expression of angiostatin in experimental CNV significantly reduces the size of CNV lesions (50).
Transplantation of Genetically Modified Iris Pigment Epithelial Cells
Submacular transplantation of autologous iris pigment epithelial (IPE) cells has been proposed to replace the damaged RPE following surgical removal of the CNV (51). The IPE is anatomically contiguous with the RPE and has the same embryonic origin. In vitro, IPE cells share functional properties with the RPE cells, such as phagocytosis, degradation of rod outer segments, and synthesis of trophic factors. However, autologous transplantation of IPE cells alone has not yet resulted in a prolonged improvement of vision in patients with AMD, potentially because the lack of expression of one or several factors that are an important part of RPE function. Semkova et al. (51) have suggested a treatment for AMD based on transplantation of genetically modified autologous IPE cells. The most significant findings are summarized as follows: First, IPE cells were readily transduced with a high-capacity adenovirus (HC-ad) vector. Second, IPE cells secreted functionally active PEDF after HC-ad–mediated gene transfer. Third, subretinal transplantation of PEDF-expressing IPE cells inhibited neovascularization in models of retinal neoangiogenesis and prevented photoreceptor degeneration.
Antiangiogenic Gene Therapy for AMD
Ocular neovascularization is a central feature of AMD and diabetic retinopathy. CNV occurring in AMD commonly causes severe vision loss in elderly patients, and retinal neovascularization resulting from progression of diabetic retinopathy is still the most common cause of new blindness in the working-aged population (52,53). Anti-VEGF therapy has been a breakthrough therapy for ocular neovascular disease, including AMD. As discussed elsewhere, monthly intraocular injections of ranibizumab or bevacizumab have been shown to be highly effective in preserving and improving vision in neovascular AMD (54–56). Limitations of ranibizumab and bevacizumab therapy include frequent injections, risk of ocular complications (primarily endophthalmitis and retinal detachment), and a possible increased systemic risk of vaso-occlusive events. As such, a longer acting therapy, with fewer injections, may be desirable. In addition, not all patients respond fully to current anti-VEGF drugs and/or monthly peaks and troughs of therapy.
Characterization of the molecular and cellular events involved in angiogenesis has led to the identification of a number of angiostatic molecules with potential therapeutic value. Preclinical and clinical evaluation of each of these is required in order to determine potential therapeutic utility. Interferon-α2a causes dramatic involution of hemangiomas and inhibits iris neovascularization in a model of ischemic retinopathy (57,58). However, multicenter, randomized, placebo-controlled trial demonstrated that patients with CNV who received interferon-α2a do not have any involution of CNV and end up with worse vision than those treated with placebo.
VEGF is an endothelial cell mitogen that plays a central role in ocular angiogenesis (59–61). Inhibition of VEGF using anti-VEGF antibodies or soluble receptors can prevent the development of experimental iris or retinal neovascularization (62,63). VEGF kinase inhibitors, that block VEGF signaling, prevent the development of retinal and CNV (64). These are among the many indicators suggesting that anti-VEGF strategies play a role in treatment of CNV.
A novel method of posttranscriptional silencing of gene expression, called RNA interference (RNAi), was discovered (65). RNAi is a conserved cellular mechanism that silences the expression of a protein in a specific and potent fashion by utilizing double-strand RNA (dsRNA) molecules that target a particular mRNA. One dsRNA can destroy and inhibit transcriptional process of hundreds of targeted mRNA by activating the RNAi mechanism. This can result in the targeted silencing of thousands of protein molecules (66). Intravitreal injection of VEGF RNAi has been shown to inhibit the growth and vascular permeability of laser-induced CNV in a nonhuman primate, but the approach has not translated successfully into human application (67).
In addition to anti-VEGF strategies, other endogenous inhibitors of angiogenesis have been also described including angiostatin (67), endostatin (68,69), antithrombin III (70), and PEDF (71). Several lines of evidence indicate that these endogenous inhibitors are likely to be well tolerated in the eye and that they may have potential therapeutic value in the setting of pathologic ocular neovascularization. However, achieving therapeutic levels of drugs or proteins in the retina is complicated by the presence of a blood–retinal barrier. The systemic delivery of proteins to the retina is particularly problematic and at this time may alternatively require repeated intraocular injections. The patient risk and acceptance of this approach must be considered in this decision. Concern regarding toxicity associated with systemic delivery increases the appeal of local delivery approaches. Gene transfer offers novel means for local delivery of therapeutic proteins to intraocular tissues.
At this time, a variety of viral vectors are under intensive investigation and hold promise for clinical ocular gene therapy. Among those currently attracting attention are adenovirus, AAV, and lentivirus vectors. The choice of a vector depends on many factors that includes but is not limited to tropism of target cells, efficiency of transduction, size of the transgene, and needs regarding latency and duration of expression. Many endogenous, as well as synthetic, factors have been shown to possess potent antiangiogenic activity, including endostatin, angiostatin, and soluble VEGF receptor inhibitors. Currently, there are two phase I clinical trials, one using an AAV vector to express a VEGF-binding protein and another using a lentiviral vector to express endostatin and angiostatin (32). Results from these proof of concept studies are not yet published.
Adenoviral Vector–Mediated Antiangiogenic Gene Therapy
Preclinical proof using either recombinant adenovectors to carry the genes encoding PEDF and endostatin or recombinant AAVs carrying the transgene encoding for angiostatin have been published and demonstrate significant inhibition of CNV in various animal models. The intravenous administration of an adenoviral construct carrying the murine endostatin gene was tested in a murine model of CNV and found almost complete inhibition of neovascular activity. Similarly, subcutaneous injection of an AAV carrying a truncated angiostatin gene resulted in significant inhibition of retinal neovascularization. These encouraging results with endostatin and angiostatin suggest a potential role of antiangiogenesis in ocular disease.
PEDF has received major attention. PEDF was first described in 1989 by Tombran-Tink (72) in conditioned medium from cultured, fetal RPE cells; it was found to be a potent neurotrophic factor. Subsequently, PEDF has been purified and cloned both from humans and mice (73). The gene is expressed as early as 17 weeks in human fetal RPE cells, suggesting that PEDF is intimately involved in early neuronal development. PEDF attracted even more attention when Dawson et al. (71) demonstrated that PEDF is one of the most potent natural inhibitors of angiogenesis. In addition, PEDF is an inhibitor of endothelial cell migration. The amount of inhibitory PEDF produced by retinal cells was positively correlated with oxygen concentrations, suggesting that retinal cell loss plays a permissive role in ischemia-driven retinal neovascularization. Moreover, a correlation between changes in VEGF/PEDF ratio and the degree of retinal neovascularization in a rat model was demonstrated.
The AdVPEDF.11 is a replication-deficient adenovirus vector designed to deliver the human PEDF gene. Intravitreous injection of AdPEDF resulted in increased expression of PEDF mRNA in the eye, compared with AdNull (the same vector without the transgene) or with uninjected controls. PDEF trail was present not only in the retina but also in other parts of the eye, including the iris, lens, and corneal epithelium. After subretinal injection of AdPEDF, it was strongly detected in the RPE cells compared with other ocular structures (73).
SAFETY AND OBSTACLES IN GENE TRANSFER
The theoretical safety concerns regarding human gene transfer are not trivial. For the individual recipient, there is the possibility of vector-induced inflammation and immune responses. There are also theoretical issues that are important to society, including concerns about modifying the human germ line and about protecting the environment from new infectious agents generated from gene transfer vectors carrying expression genes with powerful biologic functions.
There have been serious adverse events in human gene transfer trials, including inflammation induced by the administration of adenovirus vectors and by administration to the central nervous system of a xenogenic producer cell line releasing a retrovirus vector (19). However, compared with the total numbers of individuals undergoing gene transfer, adverse events have been rare and have been related mostly to the dose and the manner in which the vectors were administered.
Two potential problems with retroviral vectors warrant discussion: insertional mutagenesis and helper virus production. Problems with insertional mutagenesis, such as activation of cellular oncogenes, are shared with any gene transfer technique that results in integration of new sequences into the cellular genome, with the possible exception of AAV. Although there are many examples of retroviral activation of cellular oncogenes in mice, these events occur in the context of a spreading infection by replication-competent virus. On the other hand, the potential for production of replication-competent (helper) virus during the production of retroviral vectors remains a concern.
No novel infectious agents generated from recombination of the transferred genome and the host genome or other genetic information have yet been detected, nor has any replication-competent virus related to the vector. Cells modified ex vivo with retrovirus vectors have been infused repetitively without adverse effects. While human gene transfer has not been implicated in initiating malignancy, the number of recipients and time of observation have not been great enough to allow definitive conclusions regarding this issue. Finally, with gene therapy used to induce production of drugs in vivo, the risk for local and systemic side effects from uncontrolled drug delivery is significant.
With the successes of the human gene transfer trials have come the sobering realities of the drug development process. Some of the following problems are generic for the field, and some are specific to the vector (19):
1.Inconsistent results: The majority of the human gene transfer studies have inconsistent results, and in some cases, the basis for them is unclear. One of the more important problems is obtaining homogeneous results in the different studies.
2.Results extrapolation: There have been several surprise examples in which predictions from gene transfer studies in experimental animals have not been borne out in human safety and efficacy trials.
3.Production problems: There are some hurdles in vector production that must be overcome before large clinical trials can be initiated. Generation of replication-competent virus is observed in production of clinical-grade retrovirus and adenovirus vectors. Lack of reproducibility, aggregation, and contamination with endotoxin also complicate production of clinical-grade plasmid liposome complexes.
4.The perfect vector: The ideal gene transfer vector would be capable of efficiently delivering one or more genes of the size needed for the clinical application. The vector would be specific for its target, not recognized by the immune system, stable and easy to reproducibly produce, and could be purified in large quantities at high concentrations. It would not induce inflammation and would be safe for the recipient and the environment. Finally, it would express the gene or genes it carries for as long as required in an appropriately regulated fashion. Clinical experience to date suggests that retrovirus, adenovirus, and plasmid–liposome vectors all need refinement. There is considerable interest in developing new vectors, but there is controversy as to which vector class is most likely to succeed, particularly for use in vivo applications.
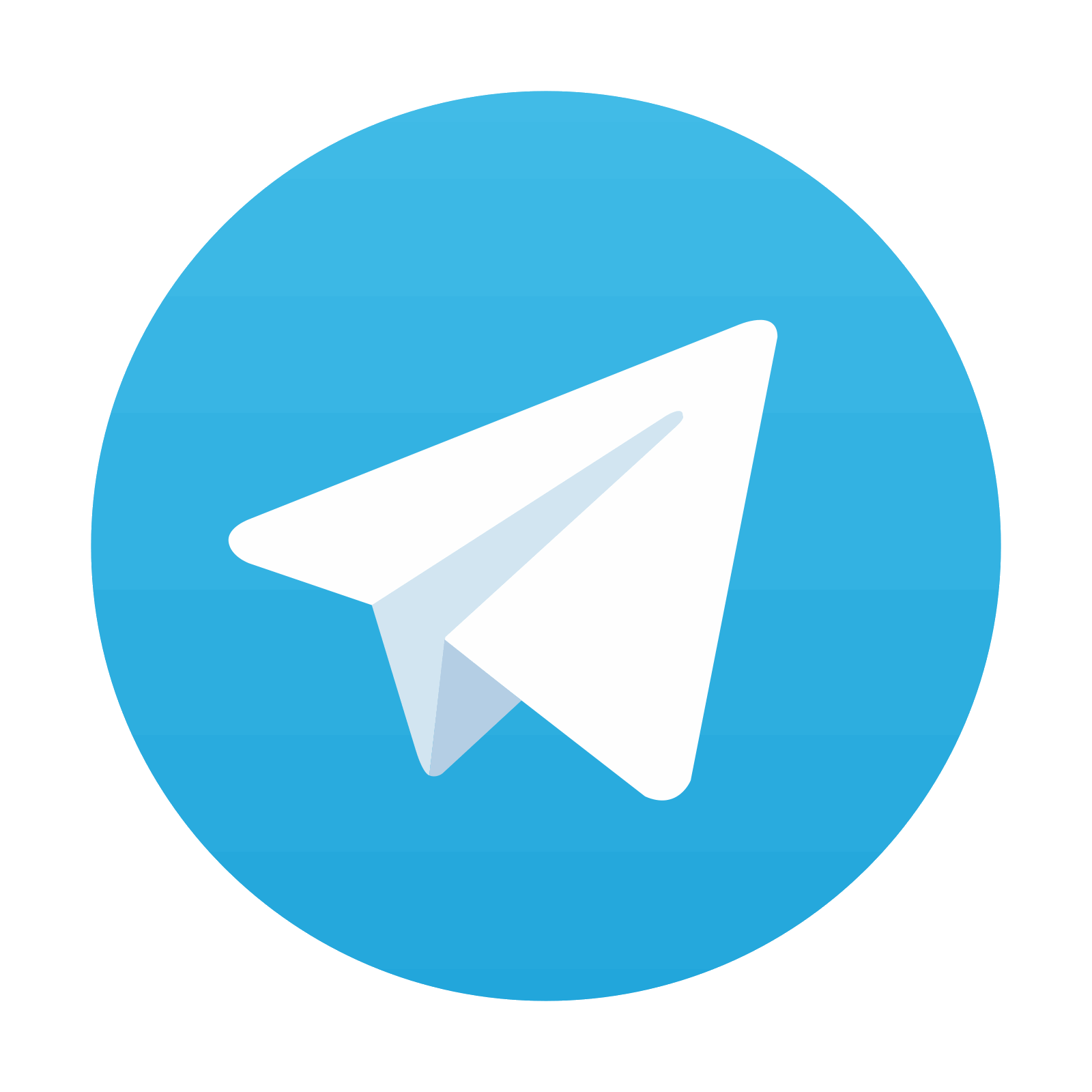
Stay updated, free articles. Join our Telegram channel
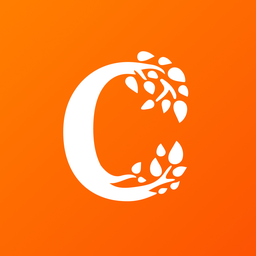
Full access? Get Clinical Tree
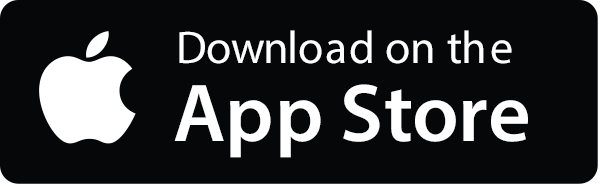
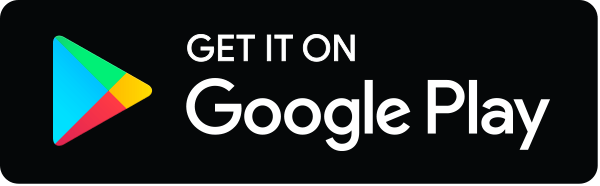