Figure 13.1 Spectral-domain OCT image demonstrating location of retinal layers in a healthy subject. Note black arrows denoting nerve fiber layer, inner plexiform layer, outer plexiform layer, external limiting membrane, RPE, and choroid. Note green arrows denoting the ganglion cell layer, inner nuclear layer, outer nuclear layer, photoreceptor inner segment/outer segment junction, and Bruch’s membrane.
Several types of treatment directed at CNV have been developed to prevent the severe visual loss in neovascular AMD patients. These include laser photocoagulation (15), photodynamic therapy (PDT) (16), and the combination of PDT with intraocular injections of triamcinolone acetonide. Despite anatomical success in some cases, there is a low chance for visual improvement when these treatments are used. In recent years, research has provided new insights into the pathogenesis of macular disease. Today, less destructive treatments directly targeting the leakage produced by CNV and its pathogenic cascade have become available (17,18). Antibodies against vascular endothelial growth factor (VEGF) uniquely offer a significant chance to improve visual acuity in patients affected by neovascular AMD. Anti-VEGF therapy can provide long-term vision maintenance in over 90% and substantial visual improvement in 25% to 40% of patients (19,20).
Monitoring response to therapy is one of the most important clinical uses of OCT. OCT can be used to quantify changes in central retinal thickness, macular volume, and subretinal fluid. These parameters, in combination with visual acuity, are used to analyze the response to therapy in several diseases including neovascular AMD. Fung et al. (21) used OCT to guide the treatment of neovascular AMD and demonstrated that OCT could detect the earliest signs of recurrent fluid in the macula after the ranibizumab injections were stopped.
This chapter discusses the OCT findings in neovascular and dry AMD, the importance of the OCT on AMD management, and the choroidal changes observed with OCT.
OCT FINDINGS IN NEOVASCULAR AMD
FA remains the gold standard for initial diagnosis of CNV, the defining characteristic of neovascular AMD. Correlation between OCT findings and leakage and staining on FA has been demonstrated using both TD-OCT and SD-OCT systems (10,14). Small changes in the structure of the retinal layers and subretinal space can readily be noted allowing the detection of morphologic changes that may signal progression or regression of the lesions. As such, SD-OCT is a useful tool in diagnosing and monitoring anatomical changes associated with neovascular AMD.
Classic CNV on OCT can be seen as a highly reflective fibrovascular tissue with irregular yet defined borders between the RPE and Bruch’s membrane or above the RPE (22). Likewise, CNV may also appear as a localized fusiform serous or fibrovascular pigmented epithelial detachment (PED) (23). CNV not well defined by FA, or occult CNV, may present as an RPE elevation from the underlying choroidal plane and may be composed of either serous or fibrovascular tissue (23,24).
CNV, when active, is associated with presence of fluid, which appears on OCT as well-circumscribed hyporeflective spaces that distort the surrounding retinal architecture. The fluid can accumulate in the subretinal space, sub-RPE space, or between all layers of the inner retina, and it can be quantitatively evaluated with OCT (Fig. 13.2) (24,25). Recent studies show that SD-OCT is superior to TD-OCT in detecting subretinal, sub-RPE, and intraretinal fluid making it better for evaluation of CNV activity (26).
Figure 13.2 Cross-sectional OCT images showing different types of CNV. A. Cross-sectional image (Spectralis; Heidelberg Engineering, Heidelberg, Germany) showing a fibrovascular PED, type 1 neovascularization (green asterisk), and the presence of subretinal fluid (green arrowhead). B. Cross-sectional image (RTVue; Optovue, Inc., Fremont, CA) showing a classic CNV, type 2 neovascularization, delineated as a nonuniform moderately hyperreflective formation above the RPE (green asterisk) and the presence of intraretinal cysts (green arrowhead). C,D. Cross-sectional images (Cirrus OCT; Carl Zeiss Meditec, Dublin, CA) of type 3 neovascularization (RAP). Green arrow (C) illustrates a retinal vessel transversing the retina toward the choroid. There is a small amount of visible subretinal fluid. In a different patient (D), infiltrating choroidal neovascular tissue (green arrow) can be appreciated overlying an irregular PED.
Freund et al. (27) proposed a more refined classification of CNV that is based on information from a variety of imaging techniques, such as combined FA/SD-OCT, high-speed SD-OCT, and indocyanine green (ICG) angiography, when necessary. This new classification system divides the neovascularization into three types with the goal of honing treatment strategies and providing better prognostic information.
Type 1 neovascularization is characterized by vessels proliferating under the RPE. With FA, it appears as occult or poorly defined CNV. With ICG angiography, it presents as a low-intensity hyperfluorescence. On OCT, it appears as localized fusiform serous or fibrovascular PED (Fig. 13.2A). Type 1 neovascularization usually presents as a fairly mature neovascular tissue that may incompletely respond to anti-VEGF therapy (27). Polypoidal choroidal vasculopathy (PCV) is included in this classification as a variant of the type 1 neovascularization.
Type 2 neovascularization is characterized by proliferation of neovascular tissue above the RPE, in the subretinal space. With FA, such CNV is described as classic. Using ICG angiography, it is usually more difficult to identify type 2 neovascularization due to the hyperfluorescence of the background choroidal circulation. SD-OCT localizes the vessels in between the RPE and the photoreceptor outer segments (Fig. 13.2B). Disruption of the inner/outer photoreceptor junction and intraretinal cystic spaces is often observed. Type 2 vessels may show a complete anatomic response to anti-VEGF therapy (27).
Type 3 neovascularization, commonly referred to as retinal angiomatous proliferation (RAP), is characterized by intraretinal neovascularization and has a distinct appearance on OCT. Imaging characteristics include sub-RPE CNV with intraretinal angiomatous change along with subretinal neovascularization and cystic change within the retina. These changes correlate with clinical and angiographic findings and are hypothesized to be caused by aberrant retinal–choroidal anastomosis (Fig. 13.2C and D) (28). Type 3 neovascularization appears to be sensitive to anti-VEGF therapy, if detected very early in its evolution (27).
Additional features of neovascular AMD are RPE tears or rips. They are known to occur in the setting of an RPE detachment both spontaneously as a feature of the disease and as the result of therapy. On OCT, a tear in the RPE is characterized by a focal defect in the RPE with scrolling at the borders along with pleating of the adjacent continuous RPE (Fig. 13.3). RPE rips are associated with the presence of a dome-shaped PED (29).
Figure 13.3 Cross-sectional Cirrus HD-OCT image of a fibrovascular PED before and after RPE rip. A. Cross-sectional image before rip. Note dome-shaped PED (green closed arrowhead) and intact RPE layer. B. Cross-sectional image after rip. Arrow denotes discontinuity of the RPE layer. Note RPE scrolling at the edge of the RPE defect and pleating RPE over the PED (green closed arrowhead). (Reprinted from Regatieri CV, Branchini L, Duker JS. The role of spectral domain OCT in the diagnosis and management of neovascular age-related macular degeneration. Ophthalmic Surg Lasers Imaging. 2011;42 Suppl:S56–66, with permission.)
Additionally, end-stage AMD can be visualized as a disciform scar made up of hyperreflective tissue in the same location as the inciting CNV accompanied by retinal atrophy (24).
ANATOMIC ASSESSMENT OF THERAPEUTIC RESPONSE IN NEOVASCULAR AMD
Currently, inhibition of VEGF-A is the first-choice therapy for neovascular AMD. Such therapy often stabilizes the vision but can result in significant visual acuity improvement in about 1/3 of treated eyes. Two of the most effective preparations, bevacizumab (Avastin, Genentech Inc. South San Francisco, CA) and ranibizumab (Lucentis, Genentech Inc.), are recombinant monoclonal antibodies (Fab) that neutralize all biologically active forms of VEGF (18). One other highly effective therapy, VEGF trap (Eylea, Regeneron, Tarrytown, NY), is a fully human fusion protein, consisting of portions of VEGF receptors 1 and 2, that binds all forms of VEGF-A along with the related placental growth factor (PlGF) (30). A fourth anti-VEGF therapy, pegaptanib sodium (Macugen, Eyetech Inc.), is available but not widely used due to its less robust clinical effectiveness (31). Two phase III clinical trials (MARINA and ANCHOR) used ranibizumab for the treatment of CNV associated with neovascular AMD (19,20). In both of these studies, ranibizumab was administered every 4 weeks (fixed schedule) for up to 2 years without monthly imaging. Both trials demonstrated prevention of substantial vision loss (lost less than 15 letters) in more than 90% of subjects. Additionally, approximately 30% to 40% of the subjects experienced substantial visual acuity gain (gain greater than 15 letters). Though these dramatic results have revolutionized the treatment of neovascular AMD, the monthly treatment schedule used in the clinical trials has a number of drawbacks including the high number of injections and the challenge of determining an end point to treatment.
Consequently, in order to evaluate the effectiveness of less frequent ranibizumab treatment schedules on neovascular AMD, the PIER study (32) evaluated a reduced-frequency treatment schedule that consisted of intravitreal injection of ranibizumab once a month for the first 3 months followed by injections once every 3 months. A reduced chance of substantial vision loss was observed. However, the treatment schedule tested failed to demonstrate an increased chance of substantial vision gain compared with sham.
As such, the best criteria for retreatment are uncertain. The strategies for treatment indication and particularly follow-up have to be adapted to new treatment modalities. Previously, when considering retreatment in neovascular AMD, FA commonly was used as an indicator of CNV activity; however, several reports have indicated inability to differentiate between leakage and staining and poor agreement in interpretation of FA in neovascular AMD between doctors, especially after treatment (10,33). Further, FA is an invasive modality rendering it not ideal for a monthly follow-up. Visual acuity alone depends on many factors such as cataract progression. It is a psychophysical measurement with limited reliability. By contrast, OCT offers many advantages: It is noninvasive and provides an objective measurement of retinal thickening and extravasated fluid. Further, OCT has been validated against FA, the gold standard, in the evaluation of retinal vascular leakage (10,13,14). OCT detects abnormalities, such as interstitial fluid, retinal cystoid abnormalities, and subretinal fluid, when fluorescein leakage from CNV is present. SD-OCT also seems to detect abnormalities frequently in the absence of fluorescein leakage from CNV. Khurana et al. (14) confirmed that the abnormalities more frequently detected on SD-OCT seemed to correspond to 90% of cases with fluorescein leakage from CNV. Additionally, studies have shown a correlation between retinal morphology on SD-OCT and visual function. For example, reduction in thickness of the neurosensory retina at the foveal center correlates with improvement in visual acuity (34). Likewise, reduction of the size of subretinal tissue on OCT has been correlated with amelioration of contrast sensitivity (35). Furthermore, disruption of the inner segment/outer segment junction (IS/OS) on SD-OCT, which is an important indicator of photoreceptor integrity, has been correlated with irreversible reduction in visual function (13,36).
In contrast with previous clinical trials, PrONTO study (Prospective OCT Imaging of Patients with Neovascular AMD Treated with Intraocular Lucentis) included macular TD-OCT features as part of the retreatment criteria (21). In this OCT-guided study, visual outcomes were similar to the results from MARINA and ANCHOR clinical trial regimen; however, the patients in the PrONTO study received an average of 9.9 injections over 24 months. In analysis of the study data after completion of the clinical trial, it was demonstrated that OCT findings predicted the need for retreatment based upon features, such as appearance of macular cysts or subretinal fluid, and these signs were thought to represent the earliest manifestations of recurrent CNV. Other OCT-guided studies using ranibizumab (37,38) or bevacizumab (39) confirmed the visual outcomes reported by PrONTO using similar numbers of intravitreous injections.
These OCT-guided studies used TD-OCT that has an axial resolution of less than 10 μm, with a relatively slow data acquisition speed (400 A-scans/second). Recently, developed SD-OCT devices offer improved image resolution of less than 3 to 7 μm with dramatically faster acquisition speeds (18,000 to 40,000 A-scan/second). Studies demonstrated that SD-OCT provides more detailed views of the intraretinal cysts, intraretinal fluid, subretinal fluid, and sub-RPE fluid, when compared with TD-OCT (13). There is a correlation between retinal morphology on OCT and visual function (34,35,40). For these reasons, SD-OCT devices have higher and earlier detection rates of CNV activity. For an average patient, earlier detection means a significant gain of vision, which may be equivalent to or better than the gain achieved with most CNV treatments (41).
The incorporation of SD-OCT into clinical practice has improved decision-making ability in the treatment of neovascular AMD (Fig. 13.4). However, these instruments have not yet been validated in the context of anti-VEGF therapy. The Comparison of Age-Related Macular Degeneration Treatments Trial (CATT) will identify the best approach to anti-VEGF therapy (bevacizumab and ranibizumab) to be used as the standard of comparison for subsequent clinical trials for neovascular AMD. This trial has four arms: two with fixed schedule for every 4 weeks and two with variable dosing schedule. On the variable dosing arm, patients are monthly evaluated using visual acuity and TD-OCT or SD-OCT images. The treatment in the follow-up will be driven by the presence or absence of fluid (subretinal, intraretinal fluid, or sub-RPE) on the OCT. This study will identify if there is any difference between anti-VEGF therapies and evaluate whether or not use of SD-OCT images can improve outcomes.
Figure 13.4 Sequential spectral-domain OCT scans of a 75-year-old woman with subfoveal CNV. A baseline scan depicted an elevation of the RPE layer, a localized fusiform thickening and duplication of the highly reflective external band (RPE/choriocapillaris complex), and intraretinal fluid corresponding to CNV. The patient was treated with intravitreous injection of ranibizumab at baseline, month 1, and month 2. Three months after the first injection, the OCT scan demonstrated improvement of macular architecture with mild intraretinal fluid. Additionally, the thickness map showed a significant decrease in the retinal thickness at the macular region. Between 3 and 12 months after treatment, three injections were administered due to the presence of discrete intraretinal fluid. It is important to note the better resolution of the 12-month scan due to image oversampling. The improvement in the resolution leads to a better visualization of retinal layers, and it is possible to note the inner/outer segment junction interruption. At 15 months from the first injection, the patient presented with intraretinal fluid and was treated with intravitreous ranibizumab. At 18 months of follow-up, no fluid was detected in the macular area, but the thickness map showed a diffuse thinning. (Reprinted from Regatieri CV, Branchini L, Duker JS. The role of spectral-domain OCT in the diagnosis and management of neovascular age-related macular degeneration. Ophthalmic Surg Lasers Imaging. 2011;42 Suppl:S56–66, with permission.)
In future studies, photoreceptor anatomy may be more readily assessed and quantified by SD-OCT and could become an important prognostic factor for patients undergoing treatment for neovascular AMD (42). Three-dimensional rendering of retinal and intraretinal structures may enable more detailed assessment of CNV, facilitating the decision-making ability in the treatment of neovascular AMD.
OCT FINDINGS IN DRY AMD
Drusen
The presence of drusen is a hallmark feature of dry macular degeneration. Conventional high-resolution fundus photography has been the traditional gold standard in the evaluation of patients with drusen and the imaging modality chosen by reading centers to follow patients in large longitudinal, epidemiologic studies (43). However, interpretation of color photographs is subject to considerable variation, and it is difficult to reproducibly outline indistinct drusen (44). Furthermore, stereoscopic images provide no direct information regarding drusen anatomy and their effect on surrounding retinal tissue and the RPE. Due to these limitations, OCT is gaining popularity in the evaluation of drusen (Fig. 13.5).
Figure 13.5 Evolution of drusen in this patient with AMD. A. The color fundus photos show subtle changes in drusen morphology. B. The high-definition OCT image illustrates drusen as homogenous elevations of the RPE with medium internal reflectivity (Cirrus OCT; Carl Zeiss Meditec, Dublin, CA). There has been a slight increase in the volume and height of the larger drusen over the interval as seen on OCT B-scan image (green arrow) and the (C) OCT-generated elevation map. New, smaller drusen have also appeared. Arrowheads denote an area of geographic atrophy as illustrated by OCT. There is loss of the outer retina and RPE and increased transmission of light into the choroid.
A descriptive study of drusen revealed 17 different types of OCT abnormalities (45). Interobserver and intraobserver reliability were high. The most common pattern revealed drusen as convex and homogenous with medium internal reflectivity. Also commonly noted are hyperreflective foci overlying drusen, which are felt to be either RPE migration or pigment (46). Of interest, it was nearly impossible to predict the ultrastructural characteristics of drusen based on their photographic appearance (45). Other possible qualitative changes on OCT include disruption of the inner segment/outer segment junction and a hyperreflective haze within the remaining outer nuclear layer, though this may simply be the unveiling of the Henle’s layer (47,48).
The photoreceptor layer overlying drusen also has been shown to be significantly thinned, with the degree of thinning directly correlated with the height of the drusen (49). It is unclear whether the anatomical changes in the outer retina are primary and precede drusen formation and RPE loss or are secondary and form as a result of alterations in their microenvironment from drusen or RPE dysfunction (49). It is also feasible that the observed retinal thinning, in some cases, is solely due to mechanical compression and that if the compressive forces are relieved, outer retinal anatomy could normalize (50). This has been demonstrated in a recent study of the natural history of drusen. Using SD-OCT and a novel segmentation algorithm, it was shown that drusen evolve dynamically overtime, growing and shrinking with an overall tendency to increase in volume and area over time (50,51).
Geographic Atrophy
Geographic atrophy is the principal cause of visual loss in 20% of patients with AMD (52). The gold standard in the assessment and evaluation of geographic atrophy has traditionally been conventional fundus photography. In the AREDS study, for example, high-resolution stereoscopic images were taken yearly and ultimately used to develop a severity scale to predict progression toward advanced AMD (53). There are limitations to this approach, however. For one, there is the nontrivial degree of intraobserver and interobserver variability in interpreting fundus photos (54). Standard photography also only renders a two-dimensional image of the retina, providing little direct information about the anatomy of the outer retina, RPE, and choroid.
Fundus autofluorescence (FAF) is another ancillary test useful in the evaluation of geographic atrophy. It relies on the fluorescent characteristics of the fluorophore lipofuscin, a toxic metabolite within the RPE. Areas of retinal pigment atrophy are devoid of lipofuscin and appear hypo-autofluorescent. A hyper-autofluorescent border may often be seen at the margins of the atrophic area. This is thought to represent swollen RPE cells filled with lipofuscin granules. These cells are at significant risk for necrosis, and this finding has been linked to progressive atrophy (55). Unfortunately, there are drawbacks to this imaging modality as well. FAF provides no direct information about the deeper retinal layers and choroid. Image quality degrades quickly in the presence of significant media opacity. The main disadvantage, however, is the poor discrimination of atrophy in the foveal and parafoveal area. Central macular pigments absorb the excitation light—necessarily making this area hypo-autofluorescent. Thus, there is not enough contrast to clearly discriminate geographic atrophy as it approaches the fovea.
Because of these limitations, OCT has taken an expanding role in the assessment of geographic atrophy. OCT systems offer several complementary imaging protocols to illustrate the extent of geographic atrophy. Patients with geographic atrophy show attenuation of the outer retina with loss of the external limiting membrane and inner segment/outer segment junction. The outer nuclear layer is thinned, and there is a posterior bowing of the outer plexiform layer (56). At the margins of atrophy, two patterns have been described: smooth and irregular (57). There is minimal outer retinal and RPE structural change in patients with the smooth margin pattern. These patients have corresponding normal autofluorescence signal at the border of atrophy. However, those patients with an irregular pattern have severe outer retinal abnormalities with significant RPE alterations at the margin. These patients have corresponding hyper-autofluorescent borders and are felt to be at risk for progressive atrophy.
Another key finding on OCT is the increased transmission of light through the retina and into the choroid. Atrophic pigment epithelium and choriocapillaris fail to scatter light, allowing deeper penetration of light into the choroid. This feature serves as the basis for the rendered OCT fundus image, which is an en face compilation of reflected light from each A-scan. Because more light is transmitted through, areas of geographic atrophy appear bright on the virtual image. Studies have shown that geographic atrophy can be measured reproducibly with these images and correlate well with measurements from FAF (58–60). Foveal sparing has been shown to be identified much more reliably and with more certainty than FAF (59). Based on OCT images, an enlargement rate (ER) of 1.2 mm2/year has been calculated (60).
The main advantage of OCT over other imaging modalities is that it provides both an en face two-dimensional view along with cross-sectional images that allow direct visualization of outer retinal anatomy. Given that visual acuity is a poor indicator of progression of geographic atrophy and that FAF does not discriminately image parafoveal atrophy, OCT will likely become the ancillary test of choice in clinical trials involving pharmaceuticals designed to delay the progression of geographic atrophy.
Geographic Atrophy Enlargement
Early attempts to determine the progression rate of GA relied mainly on serial fundus photography. In 1989, Schatz and McDonald (61) reported a mean ER of 139 μm/year in the horizontal direction. The Beaver Dam Eye Study also looked at total growth in GA over a 5-year period. They found that all of their study eyes showed some progression of GA and that the mean overall increase was 2.5 disk areas or 6.4 mm2 (61). The AREDS study similarly found an ER of 1.78 mm2/year and a mean change of 7.45 mm2 (62). FAF has also been used to assess progression, and in a study by Holz et al., a median ER of 1.52 mm2/year was found. As discussed previously, OCT can also reliably measure the area of geographic atrophy, and, as such, it can be utilized to calculate an ER. Using OCT, Yehoshua et al. (60) found an ER of 1.2 mm2/year. This ER initially seemed to correlate with baseline lesion area, but after a square root transformation of lesion area was performed, the correlation no longer existed (Fig. 13.6). It is unclear why their growth rate as measured by OCT is slower than rates previously published, but it is possible that it is due to the more precise measurements of area as rendered by OCT.
Figure 13.6 Geographic atrophy analysis. A. Sub-RPE illumination map shows an enlargement of the geographic atrophy area from January 2010 to November 2011. B. Table shows the analysis made by the Cirrus HD-OCT software regarding the atrophy area and distance from the fovea.
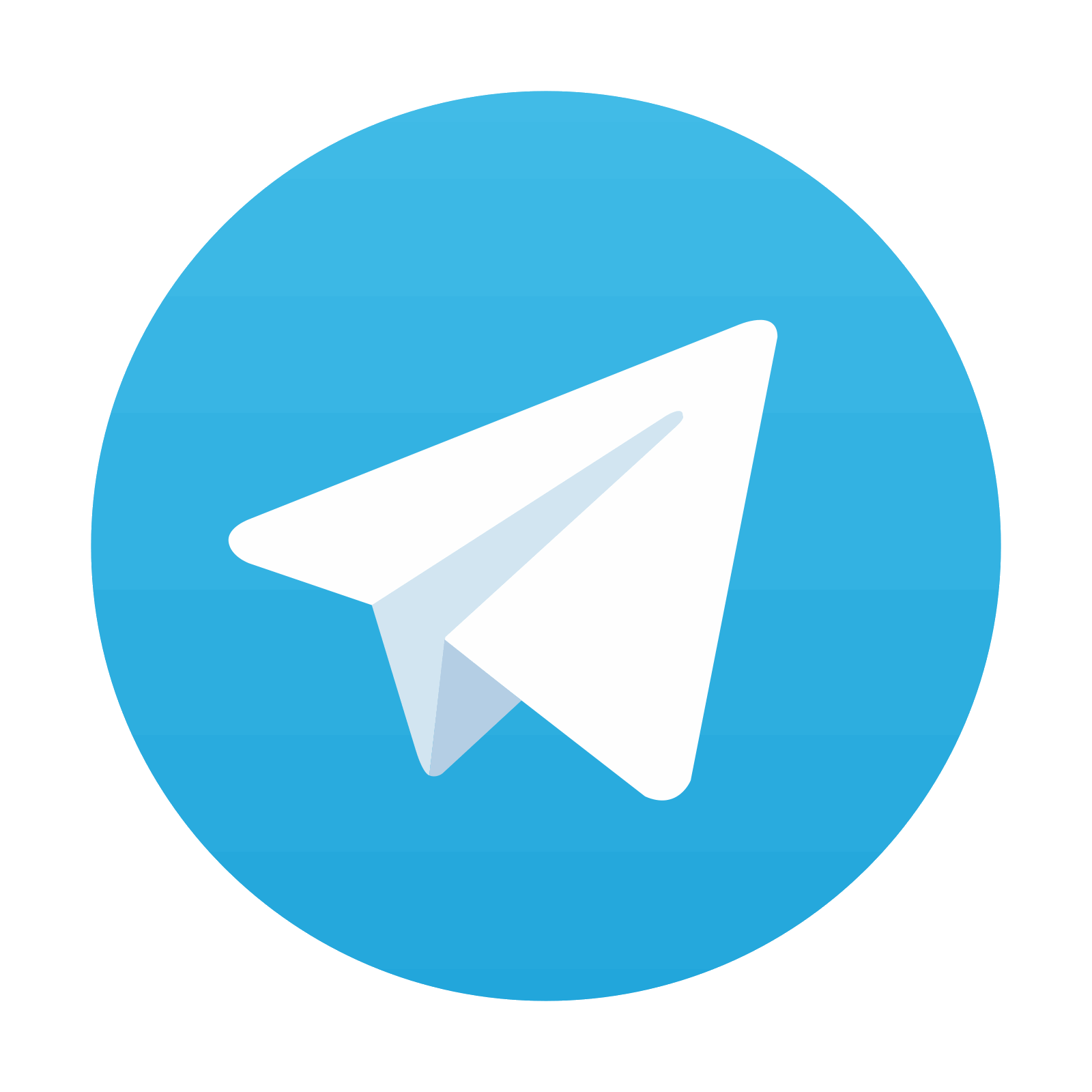
Stay updated, free articles. Join our Telegram channel
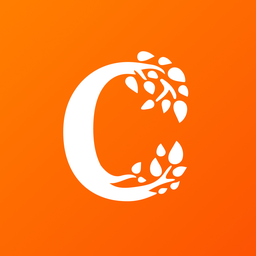
Full access? Get Clinical Tree
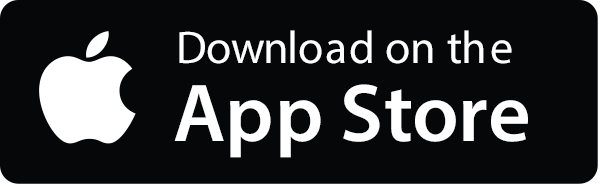
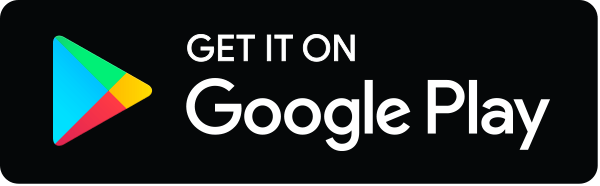