, Wayne Waltzer1 and Zhangqun Ye2
(1)
Stony Brook University, Stony Brook, USA
(2)
Tonji Medical College and Affiliated Hospital, Wuhan, Peoples’ Republic of China
78.1 Introduction
Clinical statistics has shown a stable prevalence of bladder cancer in recent years, which by far remains among the most common types of malignancy in the USA. With smoking as the most well-established risk factor, bladder cancer is the fourth most common cancer occurrences in male population [1]. In the year of 2014, an estimated 74,690 new cases are expected to occur with estimated 15,880 deaths. Bladder cancer often refers to transitional cell carcinoma (TCC) as it originates primarily from the epithelial cell layer (i.e., urothelium) of the bladder. Unlike prostate-specific antigen (PSA) for prostate cancer screening, there is currently no effective screening technique approved or recommended for the population at average risk [2–5]. As a result, hematuria (i.e., blood in the urine) is often the first clinical symptom of bladder cancer. Fortunately, urinary bladder is more accessible than prostate glands endoscopically; thus cytology following white-light cystoscopy has been the gold standard for current clinical detection of bladder cancer. This is important because bladder cancer if diagnosed prior to muscle invasion (e.g., superficial or at <pT2 stages) is curable by transurethral resection (TUR) following intravesical therapy [6]. Therefore, noninvasive early detection is crucial for the management of bladder cancer patients.
Early diagnosis of TCC, in particular, carcinoma in situ (CIS) of the bladder remains a clinical challenge [7, 8]. Although advanced molecular test such as urine cytology [5, 6], fluorescence in situ hybridization (FISH) [9], and bladder tumor antigen (BTA) [10] may provide high sensitivity and specificity in detecting high-grade TCC, it is insensitive to low-grade TCC, resulting in an overall 28–50 % of sensitivity. Additionally, as the cancer cells detected in the urine specimen can be from anywhere in the urinary tract, this technique is not capable of locating the cancerous lesions. On the other hand, due to their resolution limitation or other technical imperfection [11], current medical imaging techniques such as intravenous pyelogram (IVP), CAT, MRI, and ultrasound fail to provide useful diagnosis except for large, high-stage invasive bladder tumors. White-light imaging or cystoscopy (i.e., WLI) via direct visual inspection of bladder epithelium (urothelium) is often performed to locate TCC with subsequent TUR of suspicious lesions of the bladder and/or biopsies to verify malignancy. WLI has proven highly efficient for detecting papillary TCC, but it is common clinical scenarios that the bladder of a patient with positive cytology appears unremarkable under cystoscopy and 80–90 % of all preclinical malignancies of bladder CIS (i.e., a type of flat but high-grade aggressive TCC) demonstrate no significant outgrowth [6]. Since current cystoscopy provides no depth resolution, conclusive diagnosis and staging of malignancy relies on random biopsy which may miss nonpapillary TCC (e.g., ∼50 % of CIS [6, 7, 12]) and lead to high false-positive rates due to difficulties in differentiating them from benign inflammatory lesions. For the same reason, in the separation of recurrent TCC from benign lesions such as necrosis, scar induced by previous TUR treatments often frustrates the urologists performing surveillance cystoscopy. Clinical surveys reveal [12, 13] that possibly because of inadequate detection by urine cytology and WLI (gold standard), bladder cancer has remained the highest recurrence rate (e.g., ∼50 %) among all cancer types and is the most expensive cancer to treat. For instance, ∼1.2 million cystoscopies are performed in the USA annually, yet ∼40 % of patients with initial superficial TCC (<pT2) eventually develop muscle invasive (i.e., ≥pT2), life-threatening TCC. Therefore, a more effective imaging technique that can see below the bladder surface at a high resolution to enable early diagnosis is highly desirable for optimizing the management of bladder cancer that may lead to a significant decrease in mortality from this disease.
With the advent of technological advances, a number of emerging optical imaging methods have shown the potential to enhance current cystoscopic procedures for the diagnosis of bladder tumor. For instance, fluorescence cystoscopy using 5-aminolevulinic acid (5ALA) and hypericin has been shown to improve the detection of bladder cancer and CIS in particular [8, 14–16]. Compared with 5ALA, hexaminolevulinate (HAL, e.g., Hexvix® in Europe and Cysview® in the USA) reduces the drug induction time from 4–5 h to 1 h before fluorescence cystoscopy. A recent multicenter clinical study in the EU and USA concluded [15, 17] a considerably improved diagnostic accuracy by comparison to WLI, e.g., up to 30 % more identification of CIS and potentially reduced recurrence rate. Despite some debates regarding its phototoxicity and relatively low diagnostic specificity (e.g., high false-positive rates due to inclusion of benign lesions that are mainly associated with inflammations of the bladder mucosa), the US Food and Drug Administration (FDA) approved Cysview as an adjunct to WLI for the detection of non-muscle-invasive papillary TCC in patients with known or suspected bladder cancer in mid-2010. Narrowband imaging (NBI) as an alternative approach has been reported [18, 19] to improve the sensitivity for TCC diagnosis by enhancing the contrast of bladder epithelium and subsurface vasculature at different depths (e.g., 415 nm blue light for shallow and 514 nm green light for deep blood vessel visualization). Although NBI also suffers low diagnostic specificity, it circumvents the need for contrast agent (e.g., Hexvix) and thus reduces the associated cost and patient discomfort. Confocal endoscopy and Raman spectroscopy have also been reported to detect bladder cancers by providing subcellular morphology [20, 21] and molecular contrast of cancer cells, respectively. Interestingly, the latter may improve the specificity by discriminating inflammatory tissue and TCC, which remains a common problem of fluorescence and NBI cystoscopy for bladder cancer diagnosis. Noteworthily, in vivo Raman spectroscopy although potentially more objective may have to be combined with or guided by other cystoscopic imaging techniques to provide both high sensitivity and specificity for bladder cancer diagnosis [21, 22].
In contrast to 5ALA fluorescence, NBI and Raman techniques that detect biochemical and molecular features of bladder cancer, optical coherence tomography (OCT) has been applied to imaging the morphological alternations of bladder caused by tumorigenesis [23, 24]. As an emerging optical technique, OCT offers the unique capabilities that enable 2-D and even 3-D cross-sectional imaging of biological tissue such as bladder wall at superb resolution (e.g., 1–10 μm axial resolution) and up to 2 mm of depths, making it a promising imaging technology for detecting various superficial tumors like bladder cancer. Since its first introduction in the early 1990 [25], OCT has been fully developed to the clinical arena (e.g., for routine ophthalmological diagnosis) [26]. In addition to its successful clinical adoption in ophthalmology, there have been active research, development, and clinical studies to explore its applications in diagnosing disorders in many other non-translucent tissues such as dental cavities, vulnerable plaques in coronary vessels, as well as cancers in the skin, oral cavity, bronchus, esophagus, lung, colon, cervix, and urinary bladder [27]. For the majorities of these applications, various miniature OCT probes (e.g., OCT endoscopes) are needed to access internal organs noninvasively or minimally invasively. Recent technological advances that may have significant impacts on in vivo OCT diagnoses of epithelial cancers include the development of laser-scanning probes for endoscopic OCT [27–29], Doppler OCT (DOCT) to permit subsurface vasculatural imaging (e.g., tumor microenvironment) [30–32], and polarization-sensitive OCT [33, 34] and contrast-enhanced OCT [35] to provide more specific diagnosis. Importantly, compared to initial time-domain OCT, spectral-domain OCT and more recent swept-source OCT render in vivo 2-D and even 3-D volumetric OCT imaging of biological tissue in real time and at higher detection sensitivity (i.e., SNR) [36, 37]. On the other hand, ultrahigh-resolution OCT (μOCT) by utilizing ultra-broadband laser (e.g., ultrashort-pulse laser) has been reported to enable in vivo subcellular imaging of biological tissue [38, 39], which may shed some light on its capability for subcellular identification of cancerous cells (e.g., cancer grading). Here, we summarize the technological development and the results from our lab and others on preclinical and clinical studies of OCT for bladder cancer diagnosis.
78.2 Methods
78.2.1 OCT Engines
Like many other OCT groups, the study of our lab on OCT diagnosis of bladder cancer has spanned the transition from early time-domain OCT (TDOCT) to recent spectral-domain OCT (SDOCT). The results presented below include those acquired from both TDOCT and SDOCT engines accordingly. Figure 78.1 depicts the schematic diagram of TDOCT (a) and SDOCT (b) setups.
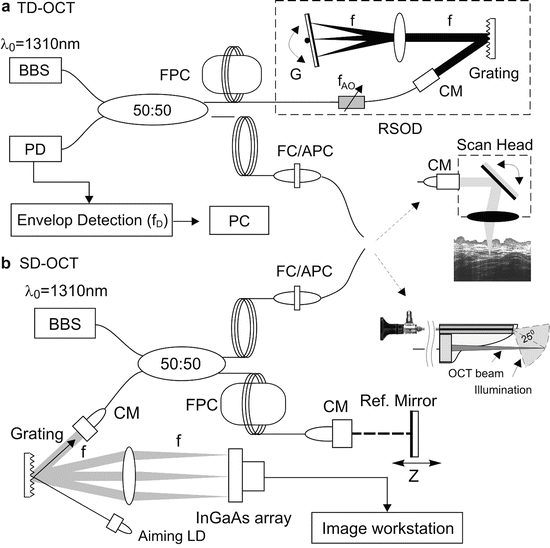
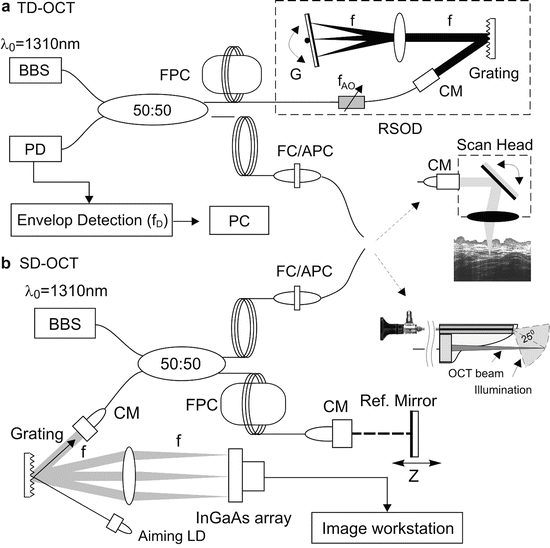
Fig. 78.1
Schematic diagrams of TDOCT and SDOCT setups. (a) TD-OCT, (b) SD-OCT. BBS: broad band source, CM collimator, FPC fiber polarization controller, G galvanometer scanner, PD photo diode, RSOD rapid-scanning optical delay line, AO differential acousto-optic modulator (e.g., fAO = 2 MHz), LD aiming red or green laser diode
For TDOCT (a), a pigtailed broadband light source whose power, central wavelength, and FWHM spectral bandwidth are I0 = 18 mw, λ0 = 1,310 nm, and Δλ = 78 nm, respectively, is employed to illuminate a fiber-optic Michelson interferometer (e.g., 2 × 2 fiber-optic coupler) which splits the light equally into the reference and sample arms and recombines the interference in the detection arm. A rapid-scanning grating-lens-based optical delay (RSOD) is used to provide axial scan (A-scan), i.e., to vary optical pathlength delay in the reference arm. As RSOD separates phase and group delays permitting independent control of Doppler frequency shift fD and bandwidth Δf of the OCT signal, a custom AO modulator is used to provide ultra-stable fD at 2 MHz (to eliminate fD drift due to scanning instability) and thus effectively enhance system SNR [40]. The sample arm is connected to either a benchtop scanning probe for ex vivo bladder characterization or a laser-scanning endoscope for in vivo bladder imaging through an FC/APC connector [29]. Typical system specifications are lateral resolution Λ = 12 μm for benchtop OCT probe and Λ = 16 μm for OCT endoscope, axial resolution Lc = 10 μm (coherence length), SNR ≈ 100 dB, and 2D image rate up to 2–4 fps (limited by the scanning rate fG ≤ 1 kHz of RSOD).
For SDOCT (b), the system is illuminated by a broader-band pigtailed source (e.g., I0 = 8 mw, λ0 = 1,300 nm, Δλ = 90 nm), providing an axial resolution of Lc ≈ 8 μm. Compared to TDOCT, it employs spectral radar to implement axial scanning, which circumvents the need for mechanical scanning (e.g., RSOD) and thus drastically enhances the A-scan rate to 7.7–92 kHz (limited by the line-scan cameras employed) [41]. In addition, the parallel detection scheme of SDOCT enables it to dramatically improve the system SNR (i.e., by a factor of N1/2 enhancement, where N is the pixel number of the spectral graph) [36]. It also allows us to easily couple a red or green aiming laser back into the fiber retrospectively through a diffraction peak (e.g., m = −1 order) of the spectral camera to instantaneously trace the region of interest under 2D/3D OCT scans. Typical system specifications are lateral resolution Λ = 10 μm for benchtop OCT probe and Λ = 12 μm for OCT endoscope, axial resolution Lc = 8 μm, SNR ≈ 112 dB, and 2D image rate up to 8–100 fps (limited by the line-scan camera, fInGaAs ≤ 92 kHz).
78.2.2 OCT Endoscopes
The combined high resolution and intermediate image depth of OCT have attributed it uniquely attractive for optically guided biopsy to permit noninvasive delineation of epithelium and the underlying morphology to detect epithelial cancers and stage their invasion. However, the development of high-fidelity laser-scanning catheters for EOCT remains a challenge. Endoscopic laser-scanning approaches that have been reported include rotary fiber with 90° microprism [27], coherent fiber bundles or rod lens endoscope [42], PZT-actuated swinging fiber [24], etc. The major engineering challenge lies in the compromise of light coupling efficiency (SNR) and beam quality (focusing capability), light scanning linearity/rate and range (FOV), and catheter size and flexibility. Typically, OCT endoscopes can be categorized to either size-view or front-view EOCT probes. 2-D and even 3-D side-view EOCT [43] suitable for imaging tubular tissue (e.g., coronary artery, esophagus, colon) has been well developed using a motorized rotary fiber joint or a micro motor together with a push-pull actuator. Front-view EOCT suitable for imaging flat tissue (e.g., oral cavity, cervix, bladder) remains a technical hurdle because it needs to be inserted into the instrument channel (e.g., 16–22Fr) of a conventional endoscope for white-light image guidance [24]. In other words, the challenge is to provide a large FOV with high lateral resolution in a slender catheter. To tackle the challenge, we have developed microelectromechanical systems (MEMS)-based cystoscopic OCT (MEMS COCT) that provides a viable solution to front-view EOCT [29]. MEMS COCT takes advantage of deep reactive-ion-etch (DRIE) CMOS-MEMS mirror technology such as small size, high speed/precision, excellent laser steering capability, and potentially low cost, all of which are crucial for high-performance endoscopic laser scanning [44, 45]. The technique has been dramatically improved to optimize the performances of EOCT (e.g., SNR, resolution, packaging, reliability) [46] and validated in ex vivo and in vivo preclinical studies as well as in vivo clinical study to examine its utility and potential limitations for bladder cancer diagnosis.
Unlike those used for optical switch, MEMS mirrors used for endoscopic applications require large actuation, large size, high linearity, and fast speed. Both electrostatic (E-ST) and electrothermal (E-TH) MEMS mirrors have been developed and tested for endoscopic laser steering. Figure 78.2 exemplifies a sketch and scanning electron micrograph of an E-ST MEMS mirror, illustrating its operating principle. The chip comprises pairs of integrated MEMS z-axis curled comb-drive micro actuators (A, B) fabricated by CMOS micromachining process, allowing for a large angular actuation (C) driven under a bidirectional sweeping voltage on two sides of the mirror. Typical angular actuation (θ) for a 1 × 1 mm2 mirror is ≤10° at 18Vp-p. The primary advantage of E-ST mirror is of high scanning speed (≤10 kHz) and precision [44].
Figure 78.3 shows an E-TH mirror driven by a bimorph hinge consisting of a stack of Al and SiO2 thin films embedded with an integrated poly-Si resistor (i.e., an E-TH actuator). Due to residual stress and difference in the thermal expansion properties between these two thin layers, the hinge curls up to an initial bending angle (θ0) above the chip plane and θ varies with the temperature (T) within the bimorph. Thus, the embedded poly-Si resistor acts as the heat source to angularly actuate the mirror, and the actuation range θ can be adjusted by parameters such as the length of the stacked hinge and the differential thermal expansion coefficient between Al and SiO2. The typical actuation angle attained is θ = 17°, providing a lateral optical scanning over 35° – surpassing the need for endoscopic OCT [29]. The resonant frequency of a 1.2 × 1.1 mm2 E-TH mirror is roughly 160–250 Hz, exceeding the speed requirement for 2D COCT. Technical issues encountered in E-TH mirrors, e.g., hysteresis, buckling, and nonlinearity, have been either eliminated or minimized to ensure high-fidelity COCT imaging [45, 47]. The lateral resolution of COCT reaches Λ = 12–16 μm, which is determined by focal length f (f = 8–10 mm) of the scan lens and beam size ϕ (eventually limited by MEMS mirror size, e.g., 1.2 × 1.1 mm2).
Figure 78.4 illustrates the packaging of MEMS-based COCT and the integration to a commercial 22Fr rigid cystoscope via its instrument channel to enable white-light image-guided OCT imaging diagnosis. Despite compromised SNR due to light loss, the first in vivo image result demonstrated the capability of MEMS COCT for delineating the morphology of porcine bladder (a close homologue to human bladder) [22].
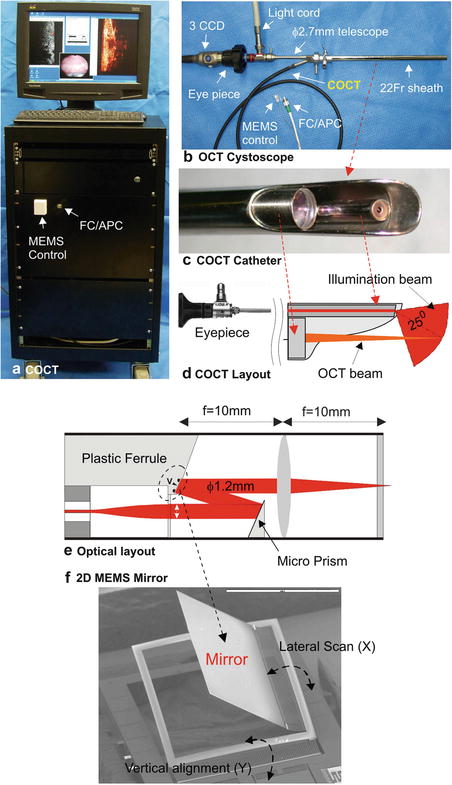
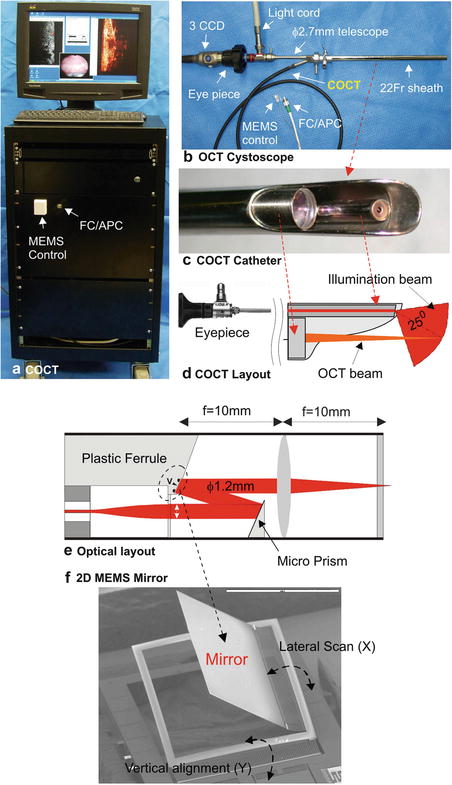
Fig. 78.4
MEMS-based COCT system. (a) A picture of the main COCT image station; (b) OCT cystoscope that fits in a 22Fr rigid sheath and connects to the main system via fiber/electric cables; (c) A picture of an COCT catheter using a ϕ2.7 mm telescope for visual guidance; (d) coordination between surface/EOCT scopes; (e) optical layout of the COCT catheter; (f) an SEM of an MEMS mirror (1.2 × 1.3 mm2) [50, 54]
78.3 Preclinical Studies
78.3.1 Bladder Morphology Imaged by OCT and Histology
Despite the contrast difference between OCT (backscattering) and histology (H&E stain), Fig. 78.5 shows the morphology of normal human bladder imaged by ex vivo OCT and the corresponding histology, indicating a close correlation for the identifications. For instance, the urothelium (U) is a thin (∼110 μm), uniform, and relatively low-scattering superficial layer; lamina propria (LP) is ∼550 μm thick and highly scattering and heterogeneous because of compositions of mostly random collagens; the upper muscularis (MS) appears largely bifurcated, likely representing the interfaces between the smooth muscle collagen bundles. This result demonstrates the resolution of OCT to delineate morphological details of human bladder and thus the potential to track their changes (e.g. tumors) so as to determine the level of invasion of early bladder cancers (e.g., to stage TA, T1, flat, or not deeply invasive) toward the bladder wall, while conventional cystoscopy often fails. It is noteworthy that although an issue for in vivo scenario, the ex vivo OCT image contrast of bladder, particularly urothelium, strongly depends on sample preparation (e.g., stretch). As the specimen was refrigerated, most of the hardened muscularis in Fig. 78.5 had to be dissected in order to stretch the bladder for OCT examination.
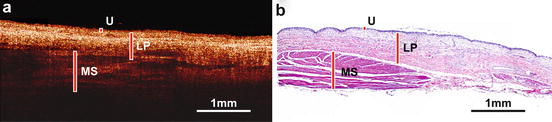
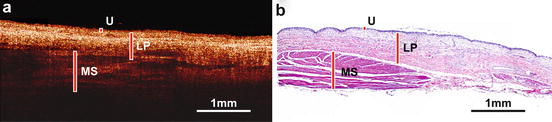
Fig. 78.5
Human bladder under proper stretch imaged by ex vivo OCT and histology, showing close correlations. Three layers in the human bladder wall critical for the diagnosis of bladder cancer prognosis, e.g., urothelium (U), lamina propria (LP), and the upper muscularis (MS), are delineated based on their backscattering differences [57]
78.3.2 Animal Bladder Carcinogenesis Imaging
OCT as a new diagnostic imaging modality must undergo throughout a validation process by a clinically proven method (i.e., histology – gold standard for cancer diagnosis) before it can be applied for human studies. In our lab, various animal TCC models have been used to systemically characterize OCT for identifications of the morphological alternations induced by carcinogenesis, which includes (1) female Fischer rats exposed to N-methyl nitrosourea (MNU) – an alkylating agent – to reproducibly induce cystitis, hyperplasia, and neoplasia (i.e., TCC) [23], (2) AY-27 cell instillation to generate multifocal TCCs in rat bladder [48], and (3) SV-40 T transgenic model to develop dysplasia, carcinoma in situ (CIS), and frank TCC in mouse bladder [49]. These protocols allowed us to define the structural and even physiological events that occurred as TCC developed in the urothelium and invaded into the underlying layers to characterize the changes in OCT images.
Figure 78.6 shows the result of ex vivo 3D OCT of rat bladder cancer (by MNU model), in which the 3-D image dataset captured in ∼6 s was reconstructed by 32 sequential 2D OCT sections with 80 μm interval. Three hyperplastic lesions and 1 TCC (stage Ta) were identified based on their backscattering differences – VU′/VU ≈ 1.07 for hyperplasia and VU″/VU ≈ 1.87 for TCC – where VU, VU′, and VU″ are the averaged backscattering values of normal urothelium, hyperplasia, and neoplasia measured by OCT, respectively. This suggests the potential role of backscattering as a natural biomarker for OCT diagnosis of early urothelial cancer primarily determined by enhanced nuclear-to-cytoplasmic ratio (NC ratio). To further study this effect, optical modeling of nuclear morphology, i.e., NC ratio changes by nuclear size, polarity, and density induced by carcinogenesis, is shown in Fig. 78.7, which collaborates with result in Fig. 78.6. In the modeling, urothelial backscattering coefficient μb was calculated using the corresponding histomorphometric data [50].
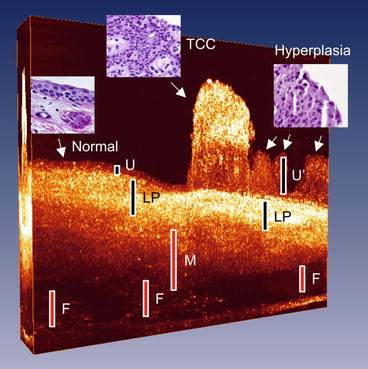
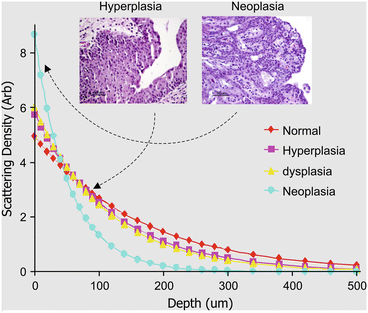
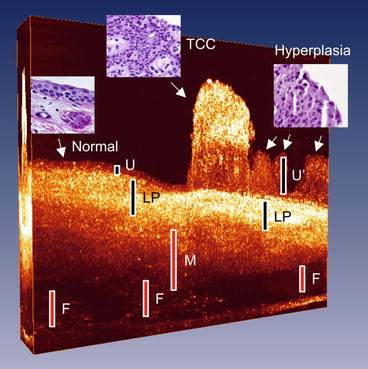
Fig. 78.6
3D OCT of rat bladder ex vivo. Image size: L6 mm × D2.1 mm × 2.5 mm, image acquisition: 6 s. White/blue dashed circles: 1 TCC and 3 hyperplastic lesions, respectively [46]
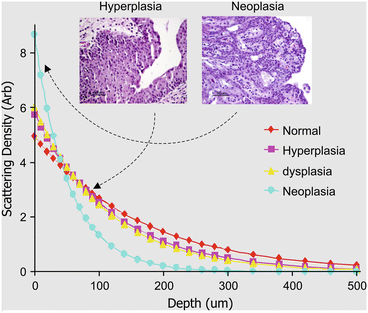
Fig. 78.7
Simulated OCT signals of urothelial backscattering versus depth during carcinogenesis by statistical evaluation of urothelial nuclear density from histological images. Normal, hyperplastic, and early dysplastic epithelia show low backscattering whereas neoplastic epithelium exhibits a ∼60 % increase in backscattering [50, 57]
In addition to diagnosis of TCC, OCT may be further used to detect bladder cancer margins. Figure 78.8 shows that 3D OCT can accurately locate the margins of a large papillary TCC. Panel 1–1′ shows that the bladder wall (e.g., U) was normal except thickened LP with excessive angiogenesis (large blood vessels, BVs). Panel 2–2′ shows the edge to TCC (stage TA) where the interface between slightly thickened urothelium U′ (210 μm) with enhanced backscattering
and the inflammatory LP was clear. Panel 3–3′ shows thick urothelium U″ (∼540 μm) with high backscattering
. OCT shows the TCC invaded into LP, but the abrupt attenuation induced by high urothelial scattering and absorption of large BVs prevents OCT to separate it from stage T1 or T2. Histology (3–3′) confirms it a T1 TCC and the lateral tumor margin (dashed red circle).
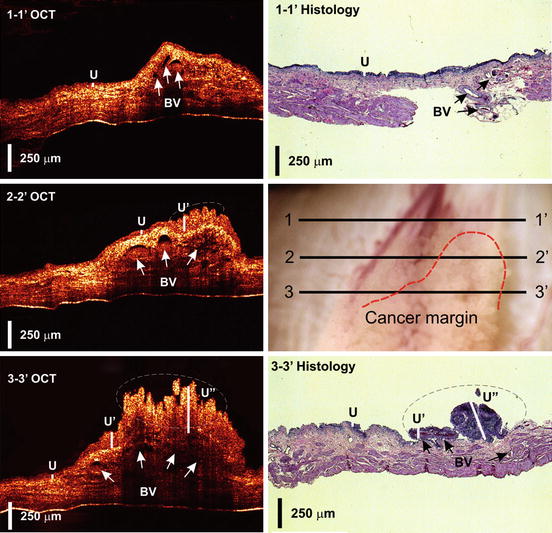
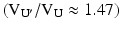
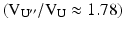
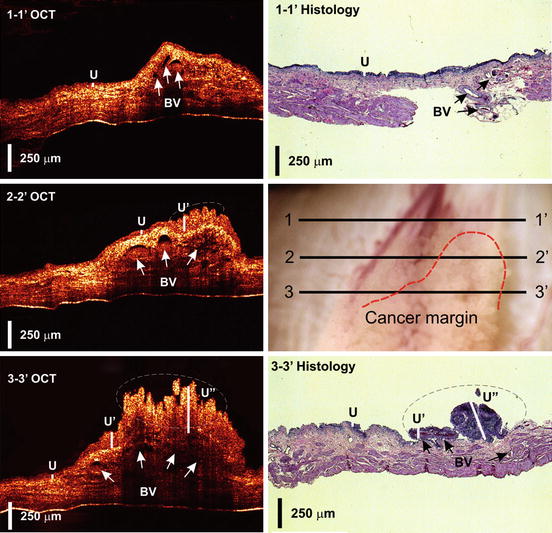
Fig. 78.8
A papillary TCC imaged by OCT and histology. Panels (1–1′) to (3–3′) were OCT sections indicated in the surface image, which covered the transition of morphological changes from “normal” to cancerous bladder areas. Corresponding histological evaluations are presented for 1–1′ and 3–3′ sections. BV: blood vessels (indicated by arrows); U, U′: normal and cancerous urothelia, respectively. White dash–circled areas: TCCs with drastically increased backscattering. Red–dashed line: precise TCC margin. The results demonstrate the potential of OCT for providing accurate precise bladder cancer margins
78.3.3 MEMS-Based OCT
In parallel to animal carcinogenesis study, preclinical study on COCT was performed to test its feasibility for clinical human examinations and to provide feedbacks for improving COCT probe design and fabrication. Figure 78.9 compares in vivo 2-D COCT image of porcine bladder (acquired at ∼6 fps) and 2-D ex vivo OCT image of almost identical bladder cross section by the benchtop setup. Except a smaller lateral size (2.9 mm vs. 6 mm) and slight decrease (∼8 dB) in image SNR due to light coupling loss in the OCT endoscope, COCT enables delineation of the morphology of pig bladder such as U, LP, and MS (identified by the interfaces between large collagen fiber bundles). Figure 78.9 demonstrates technological advances in MEMS-based COCT that allows providing comparable sensitivity and resolution to benchtop OCT; therefore, the results of ex vivo studies including animal TCC studies are potentially transferable to in vivo COCT studies [46].
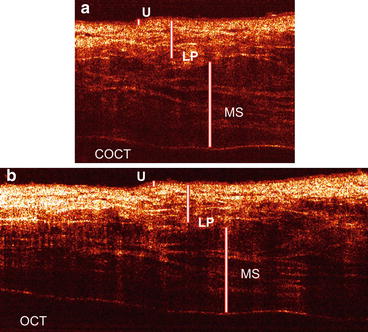
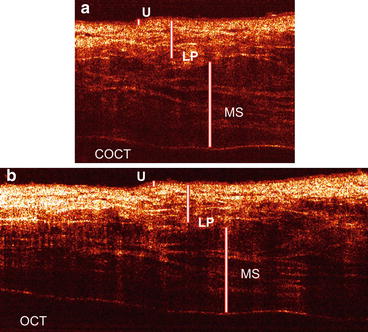
Fig. 78.9
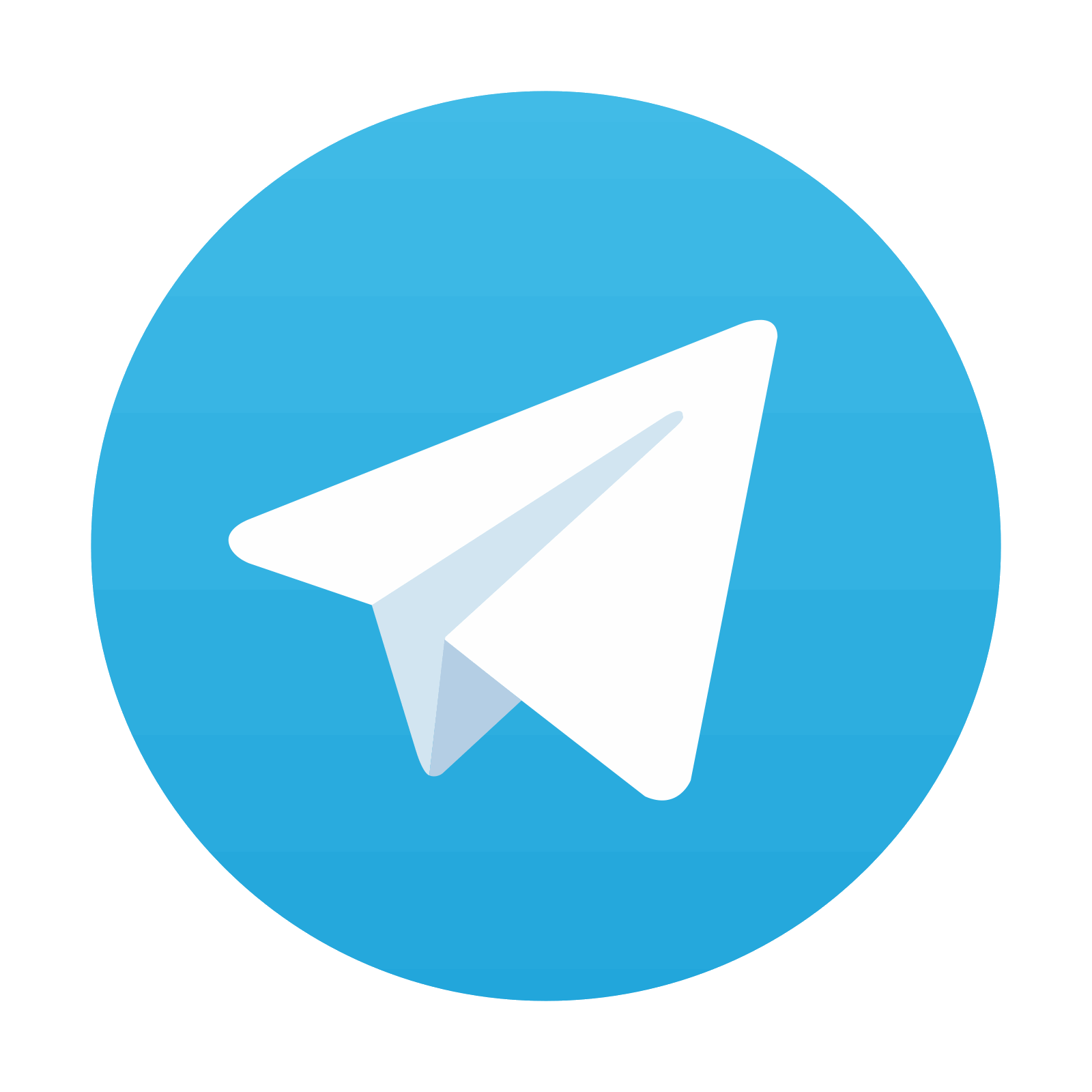
Fresh porcine bladder imaged by COCT (a) and benchtop OCT (b). Except a slight decrease in SNR in COCT, both clearly delineate bladder morphology from urothelium U to muscularis MS [46]
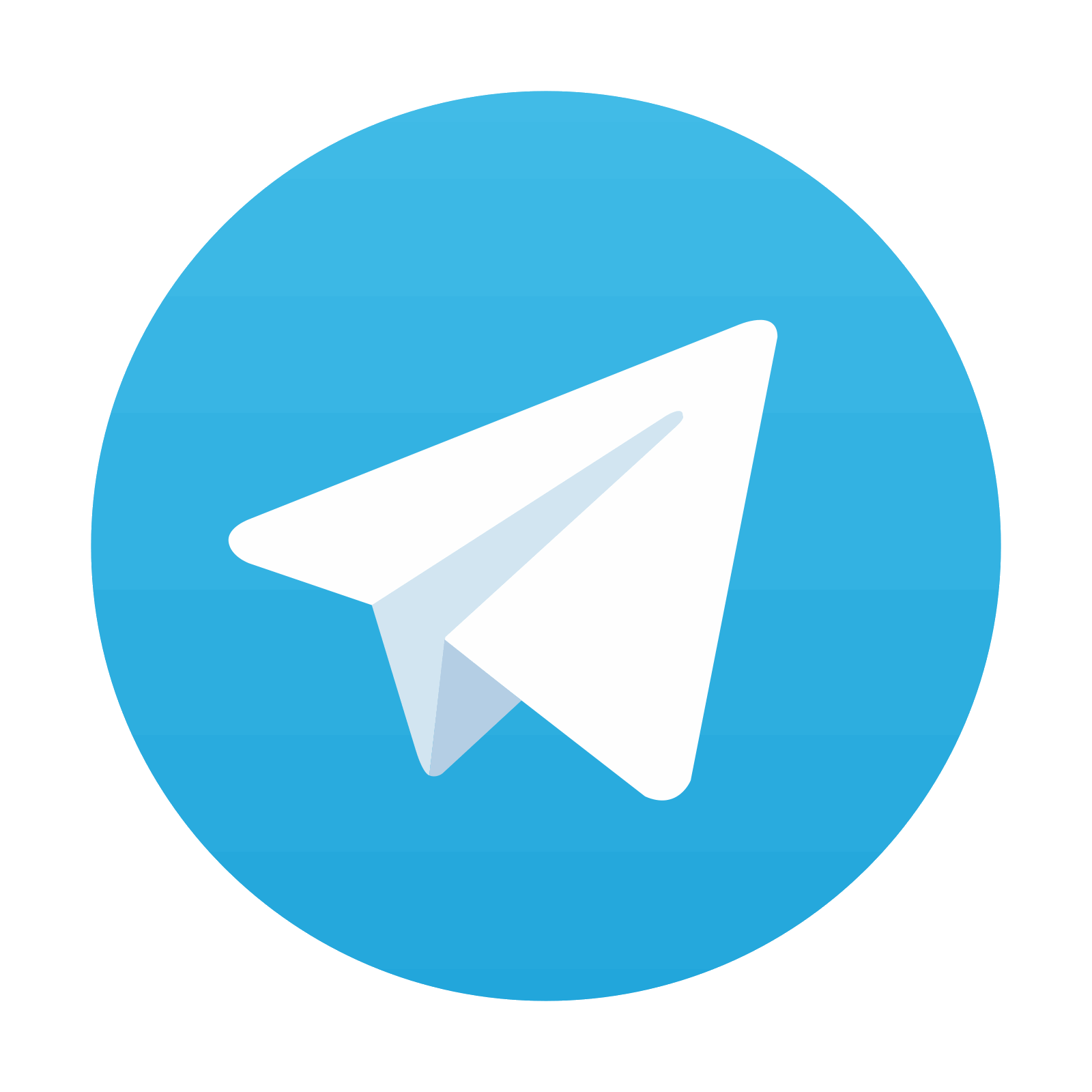
Stay updated, free articles. Join our Telegram channel
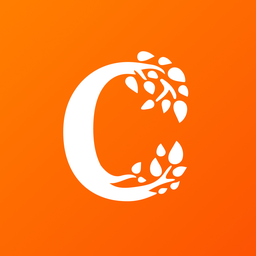
Full access? Get Clinical Tree
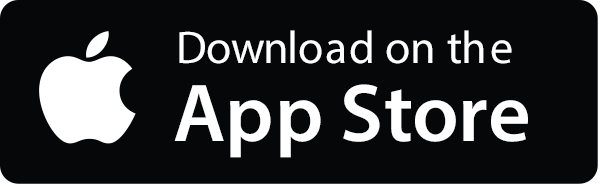
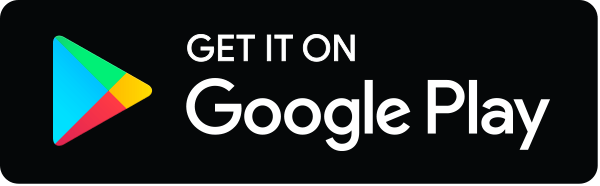
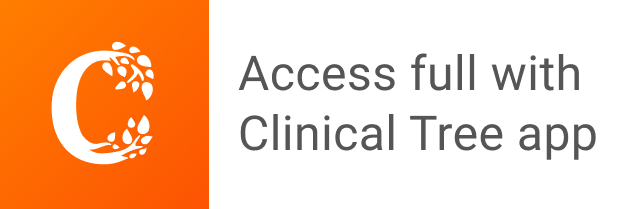