Fig. 68.1
(a) Schematic of endoscopic OCT probe with 1.8 mm diameter. (b) Photo of the OCT probe in the working channel of the endoscope. c Endoscopic view of OCT probe in human esophagus
The first demonstration of in vivo endoscopic OCT was performed in 1997 [2]. This study demonstrated OCT imaging of the gastrointestinal and pulmonary tracts in the rabbit using a 1 mm diameter fiber optic catheter and suggested the potential for internal body OCT imaging using noninvasive or minimally invasive devices. Early endoscopic OCT imaging studies of the human gastrointestinal (GI) tract were performed by several groups. Studies were performed in the esophagus and stomach [3–9], small and large intestine [5, 7, 10–12], and bile duct [13, 14]. Barrett’s esophagus was clearly differentiated from normal esophageal mucosa, and esophageal adenocarcinoma could also be distinguished from nonneoplastic tissues. Endoscopic OCT was also shown to provide complementary information to endoscopic ultrasound for staging and tumor resection [10, 15]. OCT was demonstrated to detect specialized intestinal metaplasia in Barrett’s esophagus patients [16, 17] and transmural inflammation in inflammatory bowel disease patients [12]. OCT was also investigated for differentiating hyperplastic from adenomatous polyps in the colon [11]. Endoscopic OCT studies have shown promise for detection of high-grade dysplasia in Barrett’s esophagus. Evans et al. reported a sensitivity of 83 % and specificity of 75 % for detecting high-grade dysplasia and intramucosal carcinoma with blinded scoring of OCT images from 55 patients [18]. Isenberg et al. reported a sensitivity of 68 % and a specificity of 82 %, with an accuracy of 78 % for the detection of dysplasia from 33 patients with Barrett’s esophagus [19]. Using computer-aided tissue classification techniques applied to OCT images, Qi et al. reported a sensitivity of 82 % and specificity of 74 % for identifying dysplasia in 13 patients [20].
With advances in laser light sources, OCT image resolutions approaching a few microns have been achieved [21–27]. Ultrahigh-resolution OCT imaging has reduced speckle size and overall improved image quality [28, 29]. The earliest ultrahigh-resolution endoscopic OCT imaging study in 2004 achieved 4 μm axial image resolution using a femtosecond Cr:Forsterite laser at 1,300 nm and demonstrated endoscopic imaging in vivo in the rabbit gastrointestinal tract [30]. Endoscopic OCT in small animals with even higher, 3.2 μm axial resolution, was demonstrated in the mouse using Ti:Sapphire laser at 800 nm [31, 32]. The first clinical ultrahigh-resolution endoscopic OCT study was performed using a prototype instrument with a Cr:Forsterite laser, surveying a cross section of pathologies including Barrett’s esophagus, high-grade dysplasia, and adenocarcinoma [29].
3D-OCT endoscopic imaging became possible using high-speed OCT with Fourier domain detection. Ultrahigh-resolution spectral/Fourier domain endoscopic OCT at 20 kHz axial scan rates with 2.4 μm axial resolution using a Ti:Sapphire laser was demonstrated in the mouse colon [31, 32]. Using swept-source/Fourier domain technologies at 1,300 nm wavelengths, in vivo 3D-OCT volumetric imaging of the porcine esophagus and artery was demonstrated at 10 kHz and 54 kHz axial scan rates in 2006 and 2007, respectively [33, 34]. Using Fourier domain mode locking (FDML) laser technology, record axial scan rates of 100 kHz were achieved and demonstrated for in vivo endoscopic 3D-OCT imaging in the rabbit [35] and human [36–42] gastrointestinal tract with an axial resolution of 7 um. These endoscopic studies demonstrate the impact of advances in OCT imaging technology on endoscopic OCT applications.
Gastroenterology is an especially relevant application for OCT because of the high incidence rates of GI pathologies, the clinical benefits of early detection, and the need for pre- and post-treatment assessment of therapies. In this chapter, we summarize recent advances in endoscopic OCT ranging from technology development, including ultrahigh-resolution OCT, ultrahigh-speed OCT, and endoscopic probe development, as well as clinical applications such as imaging GI pathology and assessment of endoscopic therapies.
68.2 Ultrahigh-Resolution Endoscopic OCT
Image resolution is one of the most critical parameters governing the performance of OCT systems, and therefore achieving ultrahigh image resolutions has been a key objective for endoscopic OCT. The axial image resolution of OCT is inversely proportional to the bandwidth of the light source and in order to achieve ultrahigh resolution, broad bandwidth light sources are required. The majority of previous clinical OCT studies were performed using superluminescent diode (SLD) light sources with axial resolutions of ∼10–15 μm. With recent advances in broadband femtosecond lasers, OCT image resolutions in the 2–5 μm range were demonstrated by our group and others [21–27].
Working in collaboration with LightLab Imaging, our group and collaborators developed an ultrahigh resolution endoscopic OCT system by adapting a commercial imaging engine to use an advanced femtosecond Cr4+:Forsterite laser light source (see Fig. 68.2). Although the complexity and cost of femtosecond light sources limits their potential for widespread clinical use, they can provide powerful performance advantages for small-scale validation studies. The system included several innovative features in OCT design to support very broad optical bandwidths necessary to achieve ultrahigh resolutions [30]. The axial resolution was ∼3.6 μm in tissue, corresponding to a 3–4× improvement over previous OCT technology. A fiber-optic probe was also developed for endoscopic OCT imaging. The probe was 1.8 mm in diameter and used an optical fiber, micro lens, and microprism which were distally actuated in either a push-pull or rotary motion to generate longitudinal or transverse OCT images at 4 Hz frame rate.
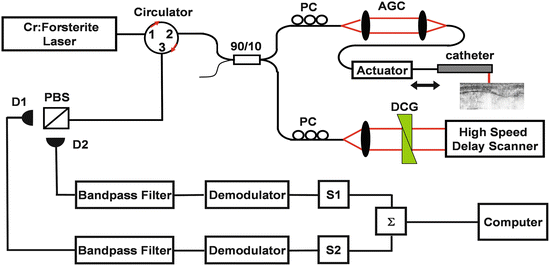
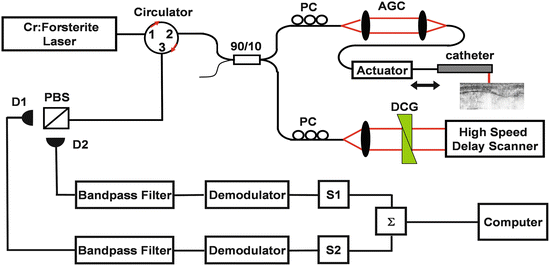
Fig. 68.2
Schematic of an early ultrahigh-resolution (UHR) endoscopic OCT system. A broadband Cr:Forsterite laser light source is used to achieve <5 μm axial image resolution. The system used time domain detection and operated at imaging speeds of 3,000 axial scans per second. The system uses a dual circulator interferometer with dual-balanced detection
Prior to clinical imaging studies, this technology was validated by performing in vivo imaging of the gastrointestinal and pulmonary tracts of the New Zealand White rabbit, correlating images with histology [30]. Figure 68.3a shows an example in vivo OCT image of the rabbit esophagus and trachea. The tracheal hyaline cartilage (hc) is visible through the esophageal wall, demonstrating the ability to achieve deep image penetration at these long wavelengths. In addition, the structural details of the tracheal mucosa and trachealis muscle are visible. The vacuous region below the tracheal wall at the bottom of the image is the tracheal airway. The corresponding histological section in Fig. 68.3b shows good correlation with the architecture seen in the OCT image. Trichrome staining was used in the histology to enhance delineation of cartilage rings in the trachea.
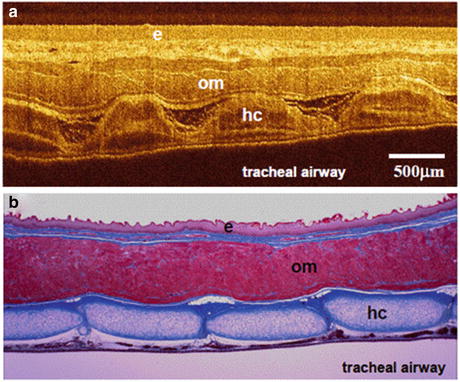
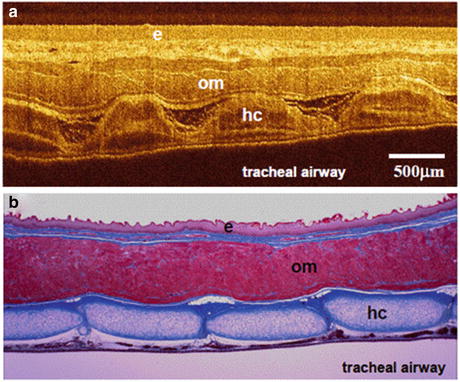
Fig. 68.3
(a) In vivo OCT image of the rabbit esophagus and trachea viewed intraluminally from the esophagus and (b) corresponding histology with trichrome staining. Tracheal hyaline cartilage (hc) between the tracheal mucosa and trachealis muscle is visualized. Note the excellent image quality which can be achieved using a broadband light source and ultrahigh-resolution OCT (From Hertz et al. [30])
Clinical ultrahigh-resolution endoscopic OCT studies were performed at the VA Boston Healthcare System [29]. Patients with previously diagnosed Barrett’s esophagus or undergoing elective colonoscopy were recruited from the endoscopy clinic at the VA Medical Center. A total of 112 patients (95 in Barrett’s esophagus group and 17 in colonoscopy group) were imaged. Imaging was performed by introducing the OCT probe into the endoscope working channel, providing simultaneous endoscopic and OCT views. Figure 68.4 shows representative ultrahigh-resolution endoscopic OCT images of the human esophagus. Figure 68.4a shows normal squamous epithelium with a characteristic layered architecture, and Fig. 68.4b shows a representative image of Barrett’s esophagus. OCT images of Barrett’s esophagus show clear differences in architectural morphology compared to normal esophageal squamous mucosa. The layered architecture is replaced by glandular structures, and low-backscattering Barrett’s glands are observed in the mucosa with interlaced regions of high-backscattering connective tissue corresponding to the lamina propria. Figure 68.4c shows a representative OCT image of high-grade dysplasia. High-grade dysplasia is characterized by irregular, distorted, and cribriform or villiform glandular architecture and is more heterogeneous than non-dysplastic Barrett’s epithelium. Figure 68.4d shows an OCT image of esophageal adenocarcinoma with epithelial disruption and stromal infiltration extending from the superficially ulcerated carcinoma. OCT images show progressive increase in architectural irregularity from Barrett’s mucosa to high-grade dysplasia and eventually to adenocarcinoma. Overall, ultrahigh-resolution OCT images showed good correlation with architectural morphology in histology (see Fig. 68.4e–h) [29]. The enhanced image resolution and reduced speckle size enabled ultrahigh-resolution OCT to visualize changes in tissue architectural heterogeneity more clearly than standard resolution OCT; however, imaging speeds were still limited compared with current technologies. In addition, although this study was the first to demonstrate ultrahigh-resolution endoscopic OCT in patients, the limited enrollment and small numbers of dysplasia cases made it difficult to identify OCT image features which could be correlated with histology and used to detect dysplasia.
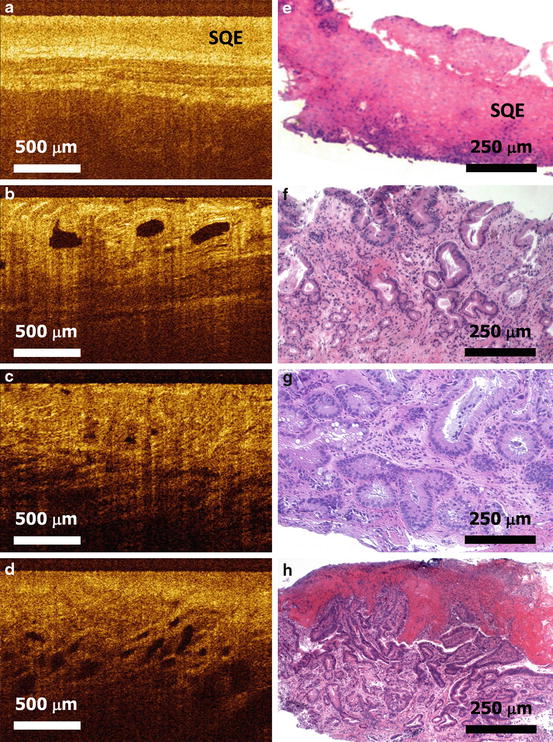
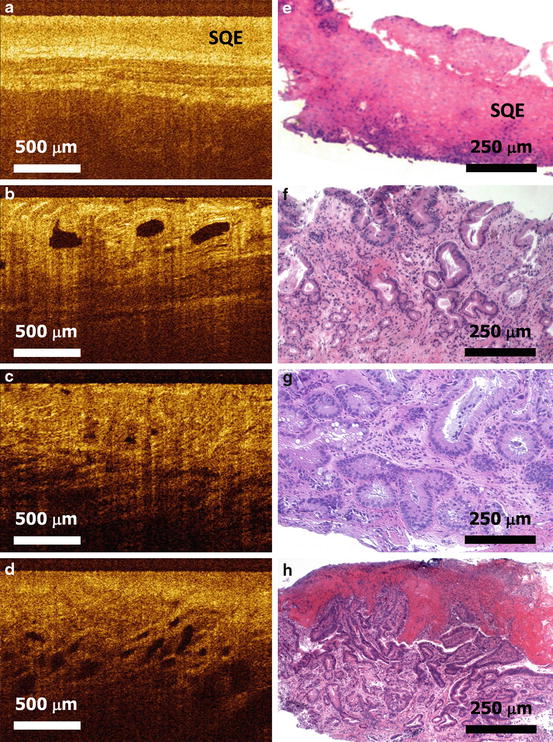
Fig. 68.4
Ultrahigh-resolution endoscopic OCT of esophageal pathology in human subjects. (a) Squamous mucosa of normal esophagus. (b) Barrett’s esophagus. (c) High-grade dysplasia. (d) Adenocarcinoma. (e–h) Corresponding pinch biopsy histology to OCT images (From Chen et al. [29])
68.3 Ultrahigh-Speed Endoscopic OCT
Recent advances in Fourier domain detection techniques enable dramatic increases in imaging speed [43–45]. These advances have had a powerful impact across multiple OCT applications ranging from ophthalmology to endoscopy. Ultrahigh speeds are important for enabling broader coverage, which is necessary for the large surface areas in the gastrointestinal tract. Ultrahigh speeds are also critical for 3D endoscopic OCT which requires the acquisition of large volumetric data sets in vivo. Swept-source/Fourier domain detection has achieved the highest imaging speeds to date and is a central focus of current research. Frequency-swept laser sources are a key technology for swept-source/Fourier domain OCT. The repetition rate of the laser sweep and the sweep range determine the OCT imaging axial scan repetition rate and axial resolution, respectively. Early swept lasers used a tunable filter with a diffraction grating and rotating polygon mirror to achieve sweep rates of 15.7–115 kHz with ∼10–15 μm axial resolutions [46, 47]. These advances in technology enabled in vivo endoscopic OCT imaging in the porcine esophagus and coronary artery at axial scan rates of 10 kHz and 54 kHz, respectively with ∼7 μm image resolution [33, 34].
68.3.1 Ultrahigh-Speed Endoscopic OCT Using FDML Lasers
The development of Fourier domain mode locking (FDML) in 2006 provided a new technique to overcame fundamental limitations in sweep rate of conventional swept lasers, enabling record OCT imaging speeds in multiple applications [35, 48–51]. FDML lasers use a long optical fiber in the laser cavity to store and regenerate the entire frequency sweep, avoiding the need to build up lasing from amplified spontaneous emission as the laser frequency is swept. This enables FDML lasers to achieve sweep rates not attainable using conventional swept lasers and to perform 3D-OCT imaging at unprecedented speeds. Higher acquisition speed enables increased field of view, high volumetric sampling densities, and reduced motion artifacts for in vivo 3D-OCT imaging. FDML lasers were used to demonstrate 3D-OCT imaging with record speeds of 370 kHz axial scan rates in 2006 [52]. In addition, FDML lasers have extremely broad tuning ranges of 160 nm at ∼1,300 nm wavelengths, supporting axial resolutions of ∼5–7 μm in tissue. Swept-source OCT using FDML laser technology enables up to a ∼50–100× improvement in speed and up to a ∼2–3× improvement in resolution compared with previous time domain OCT technology.
Working in collaboration with LightLab Imaging, our group and collaborators developed a prototype endoscopic OCT instrument using an FDML laser at ∼1,310 nm. Figure 68.5 shows a schematic of this system. The system used a dual circulator interferometer geometry for maximum signal collection efficiency combined with a dual-balanced detector. A Mach Zhender interferometer and dual-balanced detector was used to measure the frequency sweep produced by the FDML laser. The Mach Zhender calibration signal is used to recalibrate the OCT signal so that it is linear in the frequency space (k-space) before it is Fourier transformed. The prototype instrument achieved axial scan rates of 100 kHz and 5–7 μm axial resolution in tissue with real-time display and capture capabilities at 50 frames per second. To validate the prototype 3D-OCT system prior to clinical studies in humans, we performed in vivo 3D-OCT endoscopic imaging studies of the rabbit gastrointestinal tract, demonstrating record endoscopic imaging speeds in 2007 [35].
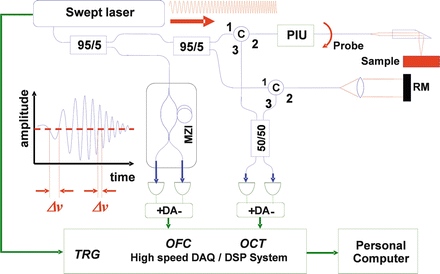
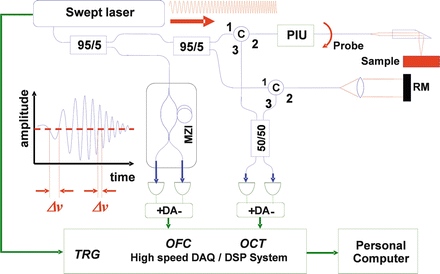
Fig. 68.5
OCT system for ultrahigh-speed endoscopic 3D-OCT imaging. The system uses swept-source/Fourier domain OCT with a high-speed Fourier domain modelocked (FDML) laser. The system uses a dual circulator interferometer with dual-balanced detection. A Mach Zhender interferometer is used to measure the frequency sweep to recalibrate the OCT signal so that it is linear in frequency or wavenumber
The increased imaging speed enabled a 9 mm segment of colon to be imaged with a high axial scan density in <18 s. Figure 68.6a shows a representative single radial OCT image of the rabbit colon with an inset showing an enlarged view of the epithelium. Colonic crypts are clearly distinguishable as dark regions surrounded by bright, filament-like bands of lamina propria. The complete volumetric data set is composed of 885 radial frames, which can be processed and displayed in three dimensions. Figure 68.6b shows a cutaway view of the rendered data set. 3D-OCT data allows virtual manipulation of tissue to facilitate visualization. Figure 68.6c shows a rectangular volume rendering, created by performing a “virtual incision” along the colon and unfolding. In addition, cross-sectional images with arbitrary orientations can be synthesized from the 3D-OCT data set. Figure 68.7a shows a longitudinal (YZ) cross section taken from near the center of the unfolded colon volume. Figure 68.7b shows an average of seven consecutive YZ slices to reduce speckle noise. Figure 68.7c shows an enlarged view of a region of Fig. 68.7b. The cross-sectional OCT correlates well with histology as shown in Fig. 68.7d although the thicknesses of the tissue layers are different in OCT compared to histology due to compression of the in vivo tissue by the imaging probe and changes in tissue from histological processing.
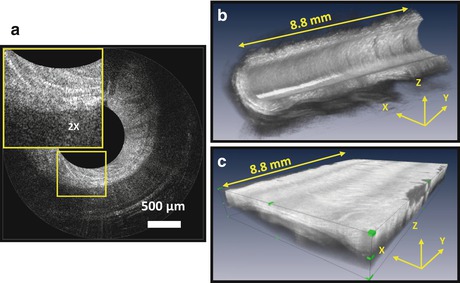
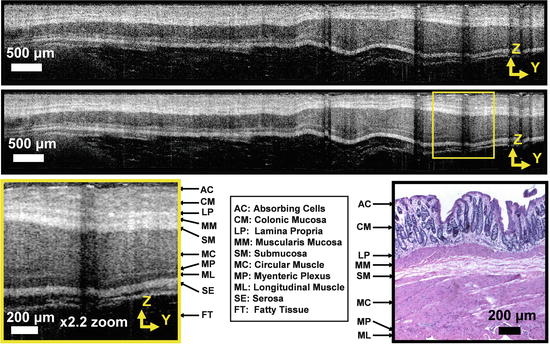
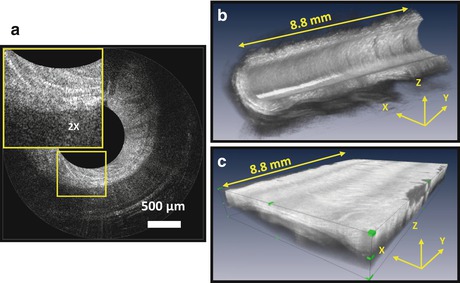
Fig. 68.6
3D-OCT endoscopic imaging at record 100 kHz axial scan rates is enabled by swept-source OCT with FDML laser technology. (a–c) 3D-OCT images of rabbit colon in vivo. (a) Single radial frame. (b) 3D cutaway rendering. (c) Unfolded volume shows tissue as rectangular slab (From Adler et al. [35])
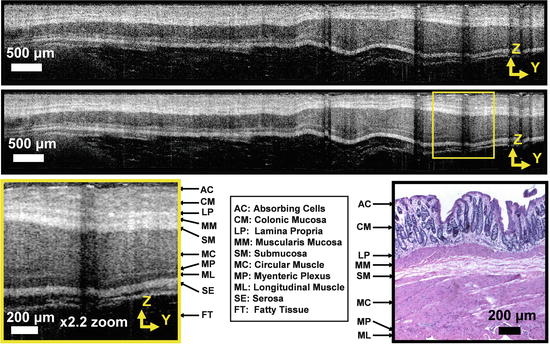
Fig. 68.7
Volumetric 3D-OCT enables the generation of arbitrary cross sections and the use of averaging to improve image quality. (a) Single cross-sectional image of rabbit colon in vivo from 3D-OCT data set. (b) Average of seven consecutive frames reduces speckle noise. (c) Enlarged view shows layered structure of colon. (d) Representative histology correlates with OCT images (From Adler et al. [35])
In order to evaluate the performance of ultrahigh-speed endoscopic OCT in clinical settings, we performed studies at the VA Boston Healthcare System [36–42, 53]. Figure 68.8 shows representative images obtained in vivo from the colon of a human subject [36]. Figure 68.8a is an en face image of normal columnar epithelial tissue formed by axial summation of the entire 3D logarithmic data set. Summation over the full axial range reduces speckle noise, while preserving only those image features that persist over significant depths. Macroscale tissue folds are visible in the mucosal surface. A mottled texture is observed due to the presence of a regular, uniform crypt pattern. Crypts are the main glandular structures in the human colon, and changes in crypt size and appearance are associated with the earliest forms of colorectal cancer [54]. The ability to assess the 3D structure of crypts is of potential value for future applications in cancer detection and treatment. Figure 68.8b shows a cross section oriented along the XZ axis, which is the fast rotational axis during data acquisition. Consistent with previous results in ex vivo human tissue [28], optical transmission is increased through the crypt lumens. Figure 68.8c shows a cross-sectional YZ plane, which is the slow pullback axis during data acquisition. Uniform crypt structures are also visible in this plane. Both cross sections were formed by averaging consecutive slices over a 20 μm thickness to reduce speckle noise. Figure 68.8d shows an enlarged region of Fig. 68.8a. The microstructural en face crypt pattern is clearly visualized and is consistent with the representative en face histology image shown in Fig. 68.8e. Figure 68.8f shows a white light video endoscopy image near the OCT imaging site. The mucosa appears regular, and the crypt pattern is difficult to distinguish by conventional endoscopy.
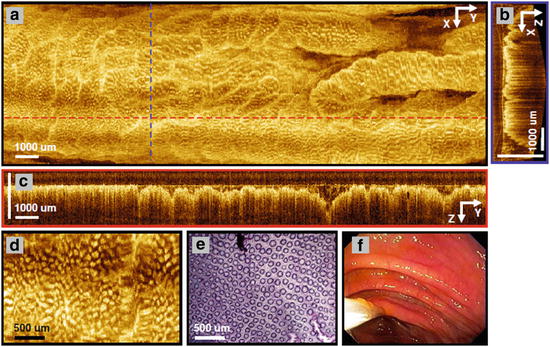
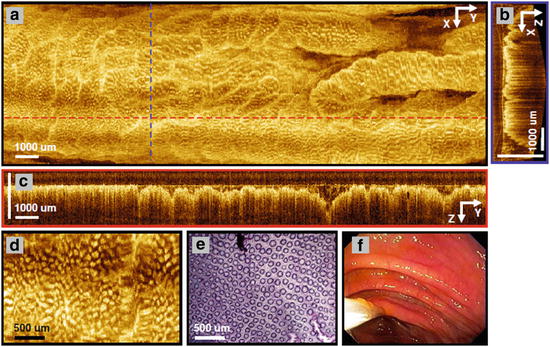
Fig. 68.8
3D-OCT images of columnar epithelial tissue in the human colon. (a) En face image constructed by axial summation of the entire data set. Dashed lines show locations of cross sections. (b) XZ cross section showing typical columnar structure. (c) YZ cross section. (d) Enlarged view of (a), showing en face crypt pattern. (e) Representative en face histology of human colon. (f) White light video endoscopy image of region analyzed with 3D-OCT (From Adler et al. [36])
68.3.2 Ultrahigh-Speed Endoscopic OCT Based on VCSEL Wavelength-Swept Light Sources
One of the most significant recent advances in OCT technology has been the development of vertical cavity surface emitting lasers (VCSEL) for swept-source OCT [55, 56]. The VCSEL operates with a single longitudinal mode instead of multiple modes and therefore has an extremely narrow instantaneous linewidth which enables a long imaging range. The micron-scale cavity length of VCSELs and the rapid MEMS response also enable extremely high imaging speeds. The VCSEL also has the advantage that both the sweep frequency and wavelength tuning range can be adjusted to support multiple operating modes with different speed, resolution, and imaging ranges. These are powerful features which make the VCSEL a promising technology for high-speed and long-range OCT imaging [57]. We developed a micromotor-based catheter with an outer diameter of 3.2 mm for ultrahigh-speed endoscopic OCT using a VCSEL swept source at a 1 MHz axial scan rate [58]. Since this study involves imaging probe technology, further details are described in the next section.
68.4 Endoscopic OCT Probe Technologies
Endoscopic probes are another critical technology for endoscopic OCT. In vivo endoscopic OCT imaging is challenging because fast optical scanning must be implemented inside a miniaturized imaging probe. Many scanning mechanisms have been investigated in catheter-based endoscopic OCT systems, such as proximally actuating a torque cable with a rotating fiber and microprism module at the distal end [2, 34, 35, 59, 60], distally actuating a fiber with a galvanometer [3], distally actuating a fiber with piezoelectric transducer [61–63], and distal beam scanning using microelectromechanical systems (MEMS) [64–67]. Imaging using proximal rotary actuation can cover a large area with a simple probe design and has been used in most endoscopic OCT applications to date. In upper GI imaging, proximal rotary scanning has been combined with inflatable balloons which serve to dilate the esophagus and stabilize the OCT probe at a controlled distance from the lumen. These methods enable dramatic improvements in imaging coverages. However proximal scanning can be unstable because the rotation is translated from the proximal end using a long torque cable to the distal imaging optics. Nonuniform rotation from torsional flexibility and friction over the torque cable length creates artifacts that limit the image quality even if the transverse optical resolution is high. The scanning speed is also limited because the torque cable becomes unstable at rotation speeds higher than a few hundred Hz. By contrast, distal scanning methods using PZT or MEMS-based actuators can provide micron-scale precision scanning because the mechanical motion is not transmitted over a long distance. However distal scanning methods are difficult to implement because the scanner must fit within the catheter.
68.4.1 Endoscopic OCT Probes Based on Micromotor Technologies
Advances in micromotor technology enable distal rotary scanning within small catheter diameters and provide large image areas while maintaining high speed and uniform rotary scanning. OCT micromotor catheters were demonstrated as early as 2004 for ultrahigh-resolution in vivo imaging of the rabbit GI tract [30, 68]. Figure 68.9 shows the schematic and photo of the imaging probe developed by our group [30]. The OCT beam was rotationally scanned by a tilted mirror actuated by a micromotor. The fiber collimator and focusing lens were attached to a cable that enables the distal focus depth to be adjusted by longitudinally translating the fiber and lens assembly. This enables the probe to be focused on structures at different depths. The assembly fits in a 4.8 mm outer diameter stainless steel housing that is enclosed within a 5 mm outer diameter transparent plastic sheath. In vivo imaging of rabbit esophagus was demonstrated at 2 frames/s with ∼3.7 μm axial and ∼8 μm transverse resolutions. Esophageal epithelium, lamina propria, and muscular mucosa can be delineated in the OCT image. Other groups have used micromotor-based OCT catheters for endoscopic upper airway imaging, studying smoke-induced injury in animal models with imaging frame rates of 20 fps [69].
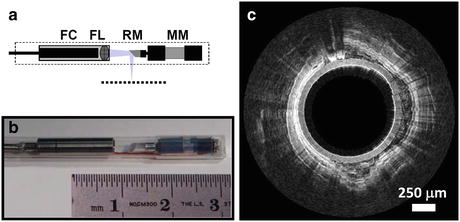
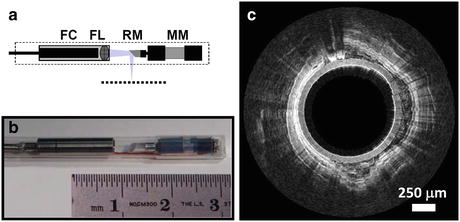
Fig. 68.9
Micromotor probes avoid the need for proximal actuation. (a, b) Schematic and photo of micromotor probe. (c) In vivo OCT image of rabbit esophagus showing normal squamous epithelial structure (From Herz et al. [30])
Although micromotor can achieve very high rotary scan speeds, early imaging studies were limited to 50 fps acquisition speeds because of the limited axial scan rates which were supported by the OCT imaging system. Using VCSEL swept-source technology described in the previous section, it is possible to achieve ultrahigh-speed endoscopic OCT using a micromotor [58]. Figure 68.10a shows a schematic diagram of a newer version micromotor-based catheter. A microprism was mounted on a 2 mm diameter, 6 mm long micromotor (Namiki Precision, Inc.). The OCT beam was focused by a fiber-GRIN lens assembly, reflected by the rotating microprism and focused outside of the catheter sheath to a spot size of 8 μm in tissue. The motor and GRIN lens were mounted inside a hypotube with a cutaway window that enables imaging over ∼70 % of the microprism rotation. A transparent plastic sheath with 2.8 mm inner diameter and 3.2 mm outer diameter was used to house the motor and optics. By pulling back the optical and motor assembly during the rotary image acquisition, a volumetric spiral scanning pattern can be generated. The micromotor drive voltage was less than 5 V and could operate at speeds from 1,200 to 72,000 rpm, corresponding to frame rates from 20 to 1,200 fps. Figure 68.10b shows a photograph of the micromotor probe. The VCEL light source operated at high 1 MHz axial scan rates and enabled high frame rates with dense sampling.
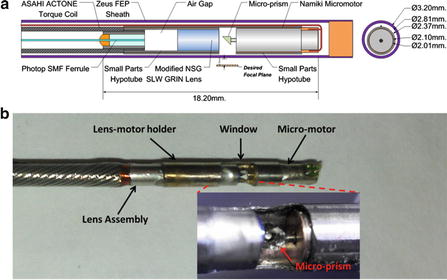
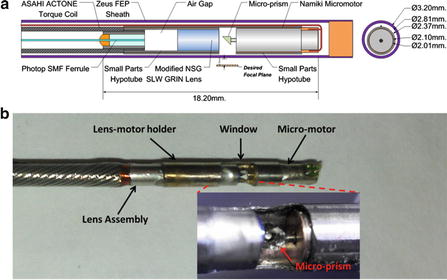
Fig. 68.10
(a, b) Schematic diagram and photograph of the micromotor probe. The probe sheath has a 3.2 mm outer diameter, and the motor and optics can be pulled back inside the sheath to generate a 3D-OCT spiral scan pattern (From Tsai et al. [58])
Figure 68.11 shows a 3D-OCT data set from the rabbit esophagus in vivo. The high-speed micromotor probe combined with the high axial scan rate of the VCSEL swept-source OCT enabled 3,000 frames of 2,500 axial scans each to be acquired in 7.5 s (i.e. 400fps), covering a volume size of 7.5 × 7.5 × 1.3 mm (x-y-z). The high axial scan rate enabled dense sampling corresponding to a pixel spacing of 4 × 2.5 × 5.2 um in the x-y-z directions, respectively. The cross-sectional OCT images (Fig. 68.11b–d) show normal esophageal layers including the epithelium (EP), lamina propria/muscularis mucosa (LP/MM), submucosa (SM), circular muscle (Ci), and longitudinal muscle (LM). The OCT images correlate well with representative histology (Fig. 68.11e). The volumetric data set could be processed and displayed in three dimensions. The images shown were generated by averaging three consecutive images perpendicular to the viewing direction in order to reduce speckle. Figure 68.11a, c shows the en face view and cross-sectional view along the pullback direction. The en face view (Fig. 68.11a) is averaged over a depth of 15 um and demonstrates the large field of view that can be achieved. Motion artifacts from cardiac motion are visible in the image. En face images are useful to differentiating features such as vasculature versus glands which can appear similar in isolated cross-sectional images.
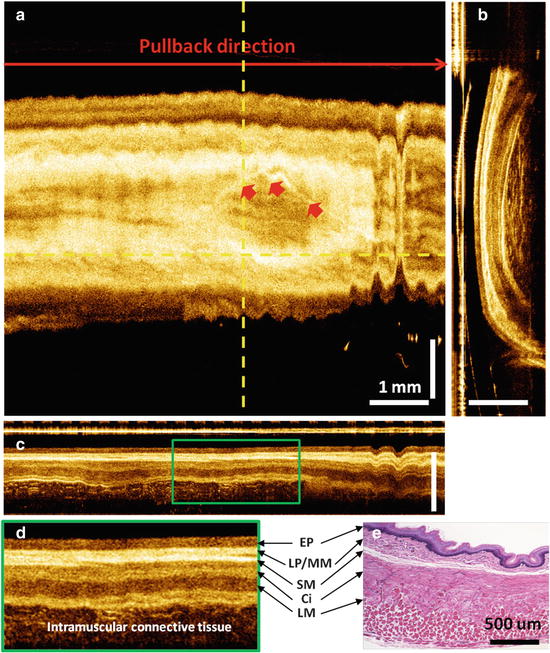
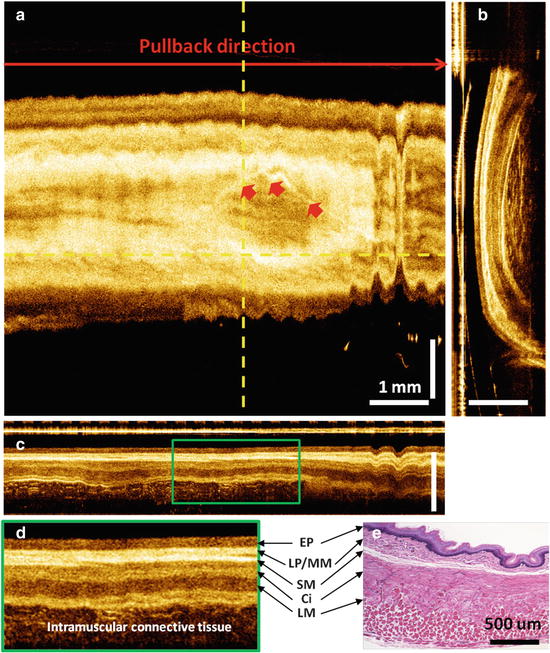
Fig. 68.11
3D-OCT volumetric images from rabbit esophagus in vivo using a micromotor probe and high-speed VCSEL swept-source OCT system. (a) En face image of the 3D data set averaged over 15 um at a depth of 150 um. (b, c) Cross-sectional images in the rotary and pullback directions. (d) Zoomed region showing detailed structure. (e) Representative histology (From Tsai et al. [58])
68.4.2 Endoscopic OCT Probes Based on PZT Technologies
High-speed endoscopic OCT probes can also be developed using other types of distal actuators such as cantilever PZT scanners [63]. Figure 68.12 shows the schematic diagram of a PZT-based probe design. A tapered 15 mm PZT bender (Piezo Systems, Inc.) was used to scan an optical fiber at its resonance frequency. A drop of epoxy (∼40 mg) was placed on the fiber tip to increase the mass and reduce the resonant frequency to ∼500 Hz. The fiber tip was angle cleaved at 8° to reduce backreflection. The scanning beam was focused with a GRIN lens objective with a microprism glued on distal end to reflect the laser beam. The spot size and scan range could be adjusted by changing the magnification. In this prototype probe, the working distance was set to be 500 μm in tissue, and the focused spot size was 20 μm, corresponding to ∼23 μm in tissue. The lateral scanning range was ∼2 mm with the PZT bender driven at 10 V (peak to peak) and a frequency of 480 Hz. The PZT bender has the advantage that the actuation voltage is very low. A thin holder mounts the PZT bender on a 2 mm outer diameter torque coil which can be pulled back from the proximal end of the probe. This produces a rater-like scan pattern for acquiring 3D-OCT data. Figure 68.12b shows the photo of the probe. The outer diameter was 3.5 mm with a rigid length of the catheter of ∼30 mm.
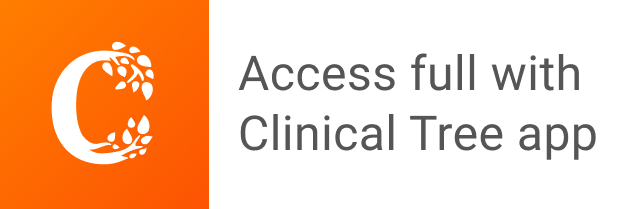