Fig. 74.1
Structure of tooth and periodontium (DeEtte M. DeVille, M.D. AMA atlas of the body: the teeth © Copyright 1999 American Medical Association)
74.1.1.1 Enamel
Consisting of 95 % mineral, 1 % water, and 4 % organic material by weight [4], enamel is the hardest structure of the body. The inorganic content of enamel consists of a crystalline calcium phosphate known as hydroxyapatite, which is also found in bone, calcified cartilage, dentin, and cementum. Although the vast majority of the enamel is occupied by densely packed hydroxyapatite crystals, a fine lacy network of organic material appears between the crystals. The bulk of this organic material is made up of the TRAP (tyrosine-rich amelogenin protein) peptide sequence tightly bound to the hydroxyapatite crystal as well as nonamelogenin proteins [4].
Because of its high mineral content, enamel is extremely hard and brittle. Ground sections of enamel reveal roughly rodlike cylindrical structures 4–6 um in diameter within the enamel, which owe their existence to a highly organized pattern of hydroxyapatite crystal orientation. For the most part, the long axes of the crystals run parallel to the longitudinal axis of the rod. This is particularly true for crystals along the central axis of the rod. Crystals more distant from the central axis, however, flare laterally to an increasing degree as they approach the rod periphery. The interrod region is an area surrounding each rod in which crystals are oriented in a different direction from those constituting the rod [4]. The capacity of OCT to image the orientation of the enamel rods and interrod region is illustrated in Fig. 74.2.
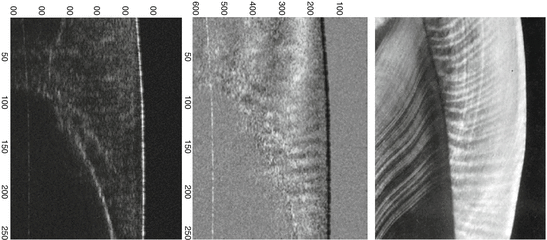
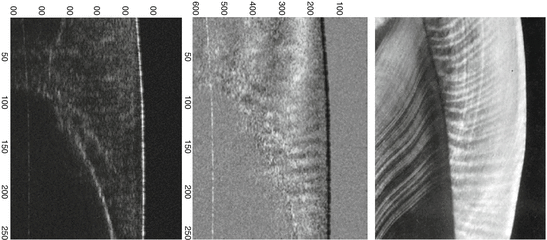
Fig. 74.2
Conventional and polarization-sensitive OCT images and a ground section of a human tooth reveal the superior capacity of polarization-sensitive OCT to image the orientation of the enamel rods and the interrod region. The junction between the enamel and dentin is characterized by a series of scallops where dentin and enamel intermix [6]
The enamel surface varies with age. In unerupted teeth, it consists of a structureless cuticle 0.5–1.5-um thick [4] overlying a 5-nm thick layer of small loosely packed crystallites interspersed with a few large platelike crystals and cuticular material [4]. This layer merges into the subsurface enamel, where 50-nm crystals are loosely packed. The subsurface layer forms the enamel surface due to rapid loss of the primary cuticle and the surface layer of small crystallites soon after eruption. An organic deposit called the salivary pellicle is present on the tooth surface, usually covered by a layer of dental plaque [4]. The important role of dental plaque in fostering dental decay will be discussed below.
74.1.1.2 Dentin-Pulp Complex
The dentin supports the enamel. The junction between the two tissues is a series of scallops where crystals of enamel and dentin intermix. Dentin and pulp are embryologically, histologically, and functionally the same tissue and are therefore considered together here [5]. Mature dentin is, chemically, by weight, approximately 70 % inorganic material, 20 % organic material, and 10 % water (adsorbed onto the surface of the mineral or in interstices between crystals). The main inorganic component is hydroxyapatite; the main organic phase is type 1 collagen with fractional inclusions of glycoproteins, proteoglycans, phosphoproteins, and some plasma proteins. Approximately 56 % of the mineral phase is within the collagen. Due to its inorganic phase, dentin is slightly harder than bone and softer than enamel [5]. Physically, dentin has an elastic quality; it is bound to the enamel at the dentinoenamel junction and covered by cementum in the root of the tooth [5].
Forming the bulk of the tooth, dentin consists of multiple closely packed dentinal tubules traversing its entire thickness and containing the cytoplasmic extensions of odontoblasts that once formed the dentin and now maintain it. The cell bodies of these odontoblasts lie along the inner aspect of the dentin, where they also form the peripheral boundary of the dental pulp [5]. The dental pulp is the soft connective tissue occupying the pulp space in the central area of the tooth, which is divided into the coronal (upper) portion, the pulp chamber, and the lower portion, the root canal. The root canals end at the apical foramen, where the main blood vessels and nerves to the pulp enter and leave the tooth. Through the apical foramen, pathologies can migrate between the pulp and the periodontium [5].
Three types of dentin are recognized in human teeth [5]. The bulk of the tooth consists of primary dentin. The structurally somewhat less regular secondary dentin develops after root formation is complete. Tertiary dentin is produced in reaction to noxious stimuli such as decay or dental procedures and is very heterogeneous in its structure. Viewed microscopically, dentin consists of several structural features. Dentinal tubules are tapered structures approximately 2.5 um in diameter near the pulp, narrowing down to approximately 900 um near the dentinoenamel junction [5]. They extend through the entire thickness of the dentin from the dentinoenamel junction to the pulp, following an S-shaped path. At their pulpal end, the tubules are generally thought to contain the process of an odontoblast bathed in dentinal fluid, although there is some uncertainty regarding the exact nature of tubule content [5]. The tubular structure of dentin permits the rapid progression of pathologies and accentuates the pulpal response to dentinal events [5]. It also allows for excellent light propagation into the pulp.
Within the dentinal tubule exits a hypermineralized (40 % more than regular dentin) ring of intratubular dentin causing a progressive reduction and the potential for eventual obliteration of the lumen of the tubule [5]. Exact mechanisms and causes are still uncertain. Intertubular dentin is located between the dentinal tubules and consists of a tightly woven network of type 1 collagen fibrils arranged roughly at right angles to the dentinal tubules, with apatite crystals deposited between and parallel to the fibrils. The ground substance consists of phosphoproteins, proteoglycans, and γ-carboxyglutamate [5]. Interglobular dentin consists of areas of unmineralized or hypomineralized dentin where zones of mineralization have failed to fuse. Here the normal architectural pattern of the tubules remains unchanged, except for the lack of intratubular dentin. The intraglobular dentin can be visualized in PS-OCT images of dentin (Fig. 74.3).
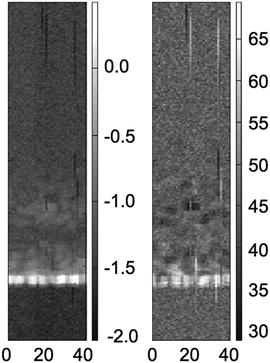
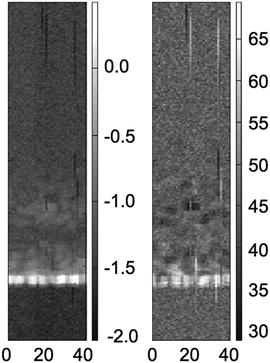
Fig. 74.3
OCT images of a human tooth near the DEJ reveal irregular masses of decreased signal intensity that were 10–20 μm in diameter and consistent with the morphology of interglobular dentin that can be seen in conventional and PS-OCT [6]
The dental pulp is the soft connective tissue that supports the dentin. Principal cells within the pulp include odontoblasts, undifferentiated mesenchymal cells, fibroblasts, macrophages, and other immunocompetent cells [5]. The major vessels and nerves lie in the central portion of the pulp. The principal collagen fibers of the pulp are type l and type lll collagen [5] in a glycosaminoglycan-, glycoprotein-, and water-containing ground substance.
74.1.2 OCT Imaging of the Hard Dental Tissues
Marked light scattering limits the transmission of visible light at 400–700-nm wavelengths through tooth substance. The relationship between light scattering in enamel and wavelength (λ) is defined as 1/λ3. At longer wavelengths exceeding 1,500 nm, light absorption by water becomes a limiting factor for the absorption of infrared (IR) light [6–8] Therefore, OCT imaging of the tooth is best performed at wavelengths between 700 and 1,500 nm, with excellent penetration to a depth of 1.5–3 mm. In 2000, Otis et al. demonstrated the superior imaging and penetration of OCT systems using a 1,310-nm vs. 850-nm light source [9].
In 1998, Colston et al. presented the first in vivo optical coherence tomography (OCT) images of dental tissue [1]. The authors presented a dental optical coherence tomography system with a very promising feature – the incorporation of the interferometer sample arm and transverse scanning optics into a handpiece suitable for intraoral use. Examples of use of this imaging system for dentistry illustrated its potential for diagnosis of periodontal disease, detection of caries, and evaluation of dental restorations. The average imaging depth of this system varied from 3 mm in hard tissues to 1.5 mm in soft tissues. Axial resolution was 15 μm (free space). The system had a lateral resolution of 50 μm and an average total lateral scan distance of 12 mm. The total scan time for each image was approximately 45 s. Further studies by this and other groups have clearly demonstrated the effectiveness of OCT for rapid, high-resolution imaging of dental tissues using OCT (please see the appropriate sections below).
Active work in the area of OCT probe development has greatly enhanced the applicability of OCT imaging for dental applications [10–20]. Figure 74.4 shows the schematic diagram and picture of a miniature probe based on a dual axis microelectromechanical system (MEMS) mirror [11]. The MEMS mirror provides high-speed, 2-axis scanning while occupying a very small volume with extremely low power consumption. The dimensions of the MEMS mirror in Fig. 74.4b are 800-mm diameter, and both axes are capable of scanning up to 30° angles at frequencies greater than 3 kHz with good linearity. The packaged probe with an outer diameter 5.0 mm or smaller can be assembled, which is sufficiently compact for dental applications (Fig. 74.4c).
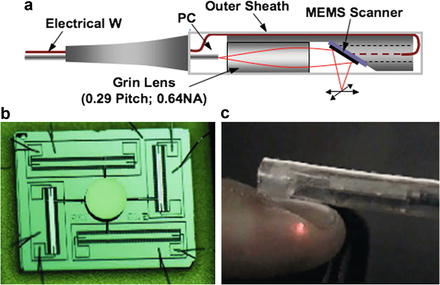
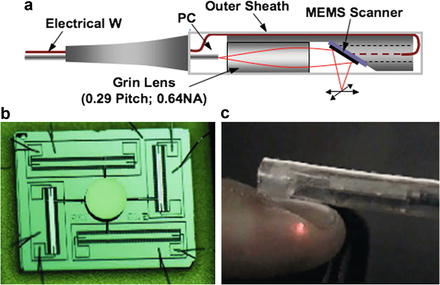
Fig. 74.4
(a) Schematic diagram of endoscopic probe; (b) photo of a vertical 2-D scanning MEMS based on comb-drive actuator. (c) photo of assembled 2-D miniature probe [11]
The 2D MEMS probe shown in Fig. 74.4c has been used for in vivo imaging of animal models and human subjects. The representative in vivo 3D images from rabbit and human tissues are shown in Fig. 74.5. These images were acquired employing the FD-OCT system, which provides adequate imaging speed for 3D imaging.
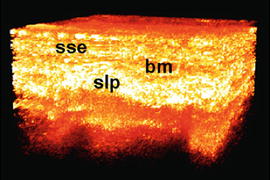
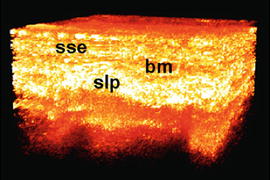
Fig. 74.5
In vivo 3D imaging of true human vocal cord obtained by a FD-OCT system using the 2D MEMS probe. Image size is 1 × 1 × 1.4 mm. Important structures, such as the stratified squamous epithelium (sse), basement membrane (bm), and superficial lamina propria (slp), are clearly visible
In addition to side-viewing probes based on MEMS, forward-viewing probes based on cantilever-type scanning have been demonstrated by several groups [19–24]. The scanning probes are driven by either PZT, electrostatic, or magnetic force [19–24]. Recently, a forward-view OCT scanning probe based on a fiber-cantilever piezotube scanner was developed by using a semi-resonant scan strategy for a better scan performance (Fig. 74.6a) [20]. A cantilever weight was attached to the fiber cantilever to reduce the resonance frequency down to 63 Hz, well in the desirable range for FD-OCT. By driving the two axes at slightly different frequencies, a low-order Lissajous pattern was obtained for a 2D area scan (Fig. 74.6b). 3D OCT images were successfully acquired in an acquisition time of 1.56 s for a tomogram volume of 2.2 × 2.2 × 2.1 mm3 (Fig. 74.6c).
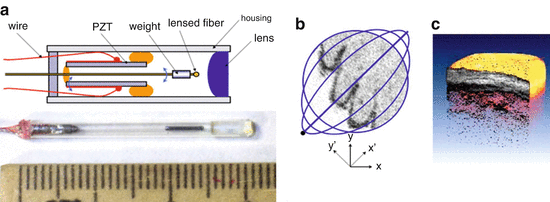
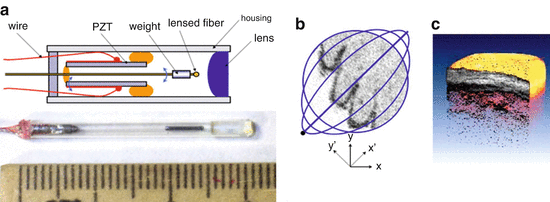
Fig. 74.6
(a) Schematic design and picture of the fabricated OCT scanning catheter. The dimensions are given in millimeters; (b) schematic Lissajous scan pattern (blue lines) in the XY plane laid over an OCT en face image; (c) the 3D-rendered tomogram [20]
These studies demonstrate the capabilities of a miniature probe for 3D OCT imaging. A 3D OCT system employing a high-speed, compact scanner may have the potential to expand the applications of OCT for dental and oral imaging and may revolutionize areas of clinical medicine as well as medical research.
74.1.3 Pathologies of Hard Dental Tissues
74.1.3.1 Decay
The most common pathological condition of the tooth is decay or caries. Cause is a confluence of cariogenic diet and inadequate oral hygiene on a susceptible tooth surface. Microorganisms multiply at the tooth surface, forming a layer of plaque. These microorganisms produce lactic and acetic acid, reducing the pH to levels as low as 3.5. At lower pHs, hydroxyapatite becomes increasingly soluble, leading to rapid demineralization and increased porosity and permeability as the calcium bound to the hydroxyapatite is ionized and washed out by the saliva. Decayed or carious lesions usually are associated with a darkening in color. However, some carious enamel lesions appear bright at the surface, especially in their early stages and are thus difficult to detect [25]. An important characteristic of the early carious lesion is that most of the demineralization occurs at the subsurface level, so that a well-mineralized surface zone remains in place for some time. This has an important implication for the early detection of carious lesions, namely, the inability to detect early lesions by clinical visual inspection of the tooth surface alone.
Diagnostic confidence also has significant impact on whether the clinician chooses to initiate further preventive therapy or restorative intervention. When caries reach the dentin, the response is sequential. Frequently at this stage, the enamel is physically still grossly intact, and bacterial invasion has not occurred. However, the lesion has increased the permeability of enamel to acid and other chemical stimuli, which now evoke a response from the dentin-pulp complex such as the deposition of tertiary dentin. Once the enamel cavitates, bacteria reach the dentin surface, and destruction of dentin begins [26].
Current Methods for Diagnosis of Caries
The technologies currently available to dental clinicians for the detection of dental caries have limited sensitivity, and there is controversy surrounding the “gold standard” for caries diagnosis. Detection of pit and fissure caries by all current methods is low. While radiographs reveal approximal lesions with moderate sensitivity and high specificity, occlusal caries are grossly underestimated in radiographic examinations [27–29]. Moreover, the widespread use of fluorides has increased the diagnostic difficulty of pit and fissure caries because enamel remineralization conceals dentinal lesions that continue to progress [29, 30]. Recently, tactile examination with a sharp explorer has been discouraged, since this may produce breakdown of the enamel surface or transfer cariogenic organisms to unaffected sites. Moreover, probing does not increase diagnostic accuracy as compared with visual examination alone [31]. Thus, visual diagnosis has been suggested as the diagnostic method of choice for pit and fissure caries [32, 33]. Trained observers detect small pit and fissure lesions by visual examination with a sensitivity of 0.6 and a specificity of 0.8. This means that 40 % of true dentinal lesions are classified as sound and that 15 % of sound surfaces are classified as diseased. In populations with a low prevalence of caries, the disease usually progresses slowly. Thus, in low prevalence caries populations, traditional diagnostic techniques worked because it is better to err toward false-negative diagnoses than to restore a sound tooth. Limited sensitivity worked in classical operative approaches, because teeth were not restored until caries involvement was significant and then the restoration was extended to include all tooth structure in the vicinity that would be considered “susceptible to caries.” Current therapies first employ preventive strategies when lesions are small and the caries risk is low. When the tooth surface is cavitated, caries significantly involve the dentin, or when caries risk is high, the diseased dentin is removed with minimal surrounding tooth structure. For today’s dentistry, the sensitivity of traditional diagnostic methods is unacceptable. The materials and operative technology permit placement of small restorations with removal of only the diseased dentin and a minimal amount of sound tooth structure. The success of these minimally invasive operative approaches depends on locating the precise region of active caries and determining with certainty that the remaining tooth structure is caries free [33]. A more precise caries diagnostic method would greatly improve the current standard of dental preventive and restorative care, with a major impact on dental health care delivery [34].
Dentists continue to rely primarily on visual and tactile examination, supported by radiographic data. However, early detection of demineralization and quantification of tooth substance loss using these techniques is extremely difficult, so that dental erosions are usually diagnosed at a relatively advanced stage when substantial irreversible enamel loss has occurred.
OCT Diagnosis of Caries
Studies indicate that OCT is a powerful technique for detecting subsurface changes in the material properties of dental tissues. OCT reliably identifies changes in dentin under sealants and adjacent to the margins of composite restorations. Comparing OCT profiles over time should allow clinicians to quickly detect potential sites of disease progression or monitor the results of remineralization therapy. Most groups have taken one of two approaches to use OCT for the detection and assessment of dental caries, using either conventional time-domain OCT or polarization-sensitive OCT.
Conventional Time-Domain OCT
Amaechi et al. [35] described the quantitative assessment of dental caries using A-scans (depth versus reflectivity curve), B-scans (longitudinal images), and C-scans (transverse images at constant depth). While the B- and C-scans qualitatively described the lesion detected, the A-scan which showed the depth-resolved reflectivity (dB) of the tooth tissue was used for the quantitative analysis. The percentage reflectivity loss (R %) in demineralized tissue, which related to the amount of mineral loss, increased with greater demineralization time. It was concluded that, using this approach, OCT could quantitatively monitor the mineral changes in a caries lesion on a longitudinal basis. In a later paper, the same group confirmed this correlation using QLF (quantitative light-induced fluorescence) [36].
A novel approach by Ko et al. combined optical coherence tomography (OCT) and Raman spectroscopy to provide morphological information and biochemical specificity for detecting and characterizing incipient carious lesions found in extracted human teeth [37]. OCT imaging of tooth samples demonstrated increased light backscattering intensity at sites of carious lesions as compared to the sound enamel (Fig. 74.7). Using Raman microspectroscopy and fiber-optic-based Raman spectroscopy to characterize the caries further, they demonstrated excellent potential for a new fiber-optic diagnostic tool enabling dentists to identify early caries lesions with greater sensitivity and specificity. In a follow-up study, Sowa et al. compared the caries diagnostic capabilities of the A-scan intensity, the OCT attenuation coefficient as well as the mean and standard deviation of the lognormal fit to the histogram of the A-scan ensemble. The OCT attenuation coefficient showed the highest discriminatory capacity. However, direct analysis of the A-scans or the histogram of A-scan intensities also showed good diagnostic discrimination [38].
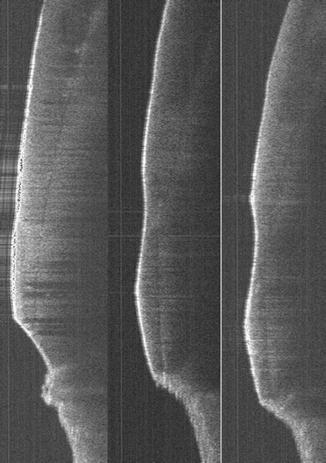
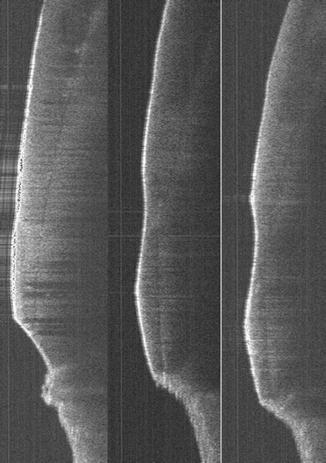
Fig. 74.7
The three OCT images show baseline enamel thickness following two five-minute exposures to a cola beverage (Otis unpublished data)
Polarization-Sensitive OCT
Birefringence of dentin and enamel provides PS-OCT with a contrast agent useful in indicating pre-carious or carious lesions [31]. PS-OCT can provide additional information related to the mineralization status and/or the scattering properties of the dental materials (Fig. 74.8). Several authors have described the use of PS-OCT for detection and diagnosis of demineralization and dental caries [41–46].
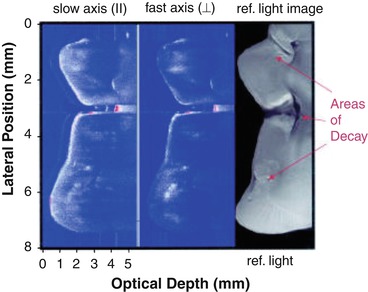
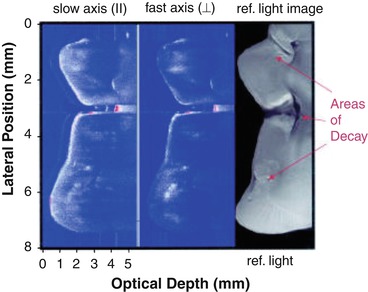
Fig. 74.8
Slow and fast axis OCT scans across the top of a third molar with occlusal decay. The reflected light image of the tooth section is shown on the right for comparison. All three carious areas are resolved in the OCT images with very strong scattering (red) in the base of the fissure shown in the center. The intensity varies from –40 to 10 dB with intensities of less than –39 dB demarcated in blue and those of intensity greater than 5 dB in red [43]
74.1.3.2 Dental Erosion, Attrition, Abrasion, and Cracking
Erosion is defined as the progressive loss of hard dental tissue by chemical processes not involving bacterial action. Repeated or prolonged exposure of teeth to acid leads to selective dissolution of specific components of the tooth surface, with eventual loss of tooth substance, hypersensitivity, caries, functional impairment, and even tooth fracture. The critical pH for enamel dissolution is 5.5. It is estimated up to 80 % of children and over 40 % of adults demonstrate substantial loss of tooth substance, an increasing proportion of which may be related to dental exposure to gastroesophageal reflux disease [47–51]. If enamel demineralization is detected sufficiently early, the enamel can be remineralized before damage becomes irreversible.
Loss by wear of tooth or restoration surface caused by tooth-to-tooth contact during mastication or parafunction is defined as attrition, whereas loss of dental tissue by wear from exogenous substances such as toothbrushes is termed abrasion. Enamel tissue loss is permanent, potentially disfiguring and can be costly and sometimes challenging to repair or mask.
Hairline cracks and fractures in the tooth tend to occur after trauma or excessive loading, especially in older patients. If these remain undetected, they can lead to pain, flawed diagnosis, and inappropriate or failed therapy.
Current Methods for Diagnosis of Dental Erosion, Attrition, Abrasion, and Fracture
The technologies currently available to dentists for the diagnosis of tooth loss include visual inspection and radiography. Diagnosis usually occurs at a relatively advanced stage (1) when substantial enamel loss has occurred, (2) damage is irreversible, and (3) differential etiological diagnosis is very tenuous. Monitoring the effectiveness of remineralizing therapies is not possible with conventional clinical tools. Diagnostic tools for cracks and fractures are limited to visual inspection and probing, neither of which is reliable. Radiographs are seldom useful.
OCT Diagnosis of Dental Erosion, Attrition, Abrasion, and Fracture
Optical coherence tomography (OCT) techniques are well suited to the quantification and imaging of enamel loss [40]. A recent publication described the use of in vivo OCT imaging to determine the effectiveness of a proton pump inhibitor to treat gastroesophageal reflux (GERD) by measuring enamel loss through erosion from OCT images [51]. The study was significant in that researchers were able to identify an association between the medication and a reduced enamel erosion vs. the control (placebo) group after a period of only 4 weeks based on the imaging data (Fig. 74.9). Several studies report on the effectiveness of OCT imaging for detecting tooth fractures and cracks, including hairline cracks in the root surface (Fig. 74.10). OCT imaging gave results compatible to far more expensive micro-CT that uses ionizing radiation. Visual observation was relatively ineffective [52, 53].
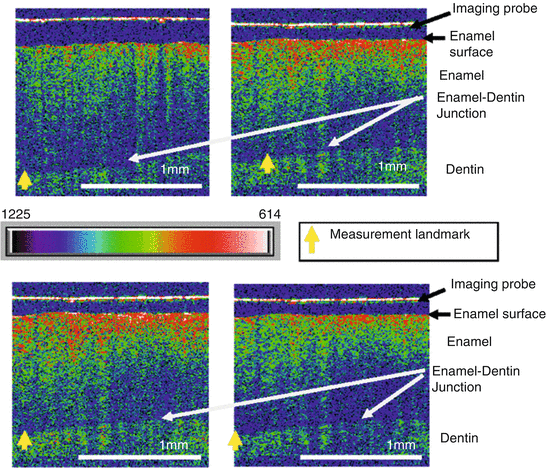
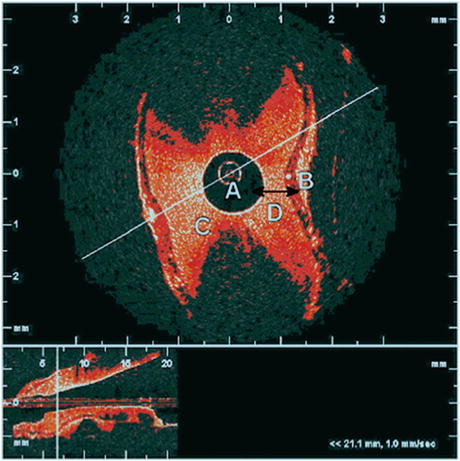
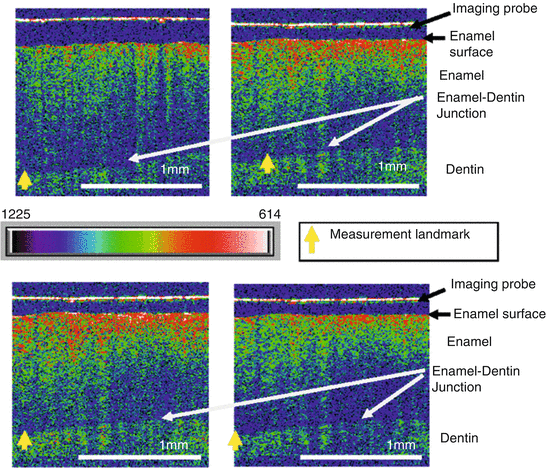
Fig. 74.9
Above: In vivo OCT images with pseudo-color superimposition of the same tooth before and after 3-week treatment with placebo. The optical signal near the tooth surface has increased as shown by the greater preponderance of green versus blue. Below: In vivo OCT images with pseudo-color superimposition of the same tooth before and after 3-week treatment with esomeprazole 20 mg bid. The optical signal near the tooth surface has decreased as shown by the reduced preponderance of red versus green [51]
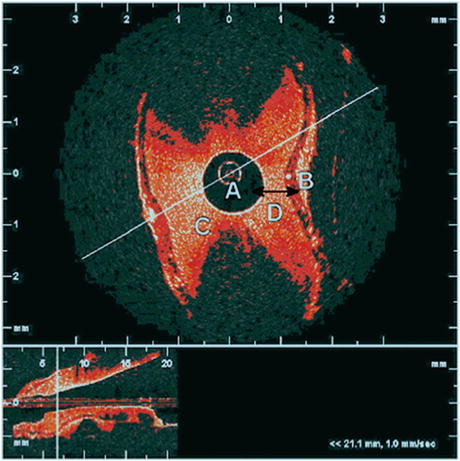
Fig. 74.10
The OCT output at 7 mm from the apex shows a cross-section of a prepared canal. (A) The root canal, (B) cementum, and (C) dentinal tubules. (D) “Risk zone” (canal wall 1-mm thick) [52]
74.1.4 Caries Prevention: Fluorides and Sealants
The most common methods of caries prevention include systemic fluoride ingestion during tooth development, topical fluoride application, especially during and directly after tooth eruption, and the use of dental sealants. Typically, sealants are placed over vulnerable areas of the teeth, especially the fissures of erupting permanent teeth, to protect them from demineralization and decay during their first few years in the oral cavity, when they are most vulnerable to demineralization and decay. Early detection of decay under sealants is important to avoid major spread of decay underneath the protective covering.
74.1.5 Diagnosing Sealant and Restoration Integrity
Radiographic evaluation and clinical examinations such as visualization and tactile probing of restoration margins are the less than adequate modalities currently available to clinicians. The capacity of OCT to image dental composite restorations offers a superior assessment as compared to radiographic imaging [54, 55]. In one study the internal characteristics of facial composite restoration were distinguished, and variations in cavosurface margins accurately identified teeth in restorations with axial cavity depths of 1, 2, and 3 mm. When the teeth were subsequently sectioned, photomicrographs revealed that OCT images depicted with fidelity the composite restorations, dentin-enamel junction (DEJ), and external tooth contours. Minimal spatial distortion of the axial wall was noted in the restorations with axial depths of 1 and 2 mm [56].
OCT has also been shown to identify accurately occlusal sealants and composite restorations [57]. In one study, 21 dentists were asked to interpret OCT images of 9 premolars that were either not restored, contained an occlusal sealant, or were restored with a composite restoration. Following a brief training period, 21 dentists evaluated the OCT images following a randomized blind protocol. The sensitivity of OCT to discriminate composite and sealants was greater than 0.92, while the specificity of discrimination was greater than 0.94. The capacity of OCT to discriminate sealants from non-restored occlusal surfaces was slightly less (sensitivity 0.88; specificity 0.86) but still within a clinically acceptable level. Inter- and intra-rater reliabilities as measured by the kappa statistic also revealed excellent performance by dentists using OCT (k = 0.82–1.0). This study also demonstrated that dentists who were previously unfamiliar with OCT images could be trained to interpret the images with a high degree of accuracy.
Not only does OCT detect sealants, it also effectively diagnoses decay underneath sealants. A study by Holtzman et al. evaluating the ability of dentists to detect decay beneath commonly used dental sealants using OCT found that, after 90 min training, dentists were able to detect tooth decay in enamel and under sealants more accurately using OCT than with visual or radiographic examination (Fig. 74.11). Detection using OCT was somewhat better prior to sealant placement than afterward. This effect varied in size depending on the type of sealant used. Radiographic diagnosis was also less accurate after sealant placement. Of the four dental sealants, Delton provided excellent positive predictive value and the best post-sealant negative predictive values [58].
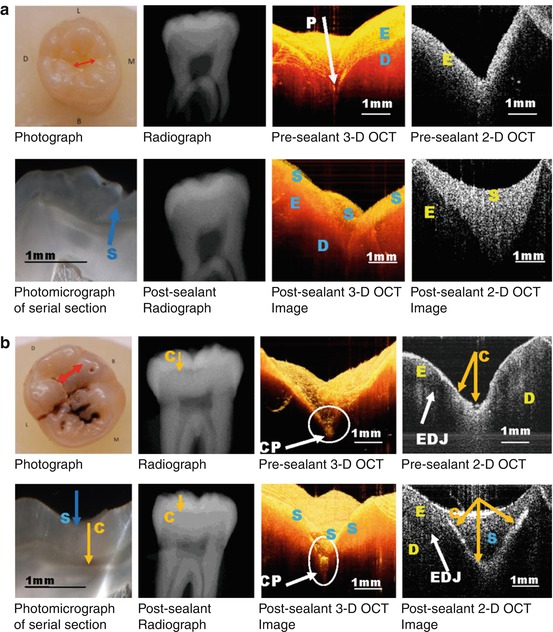
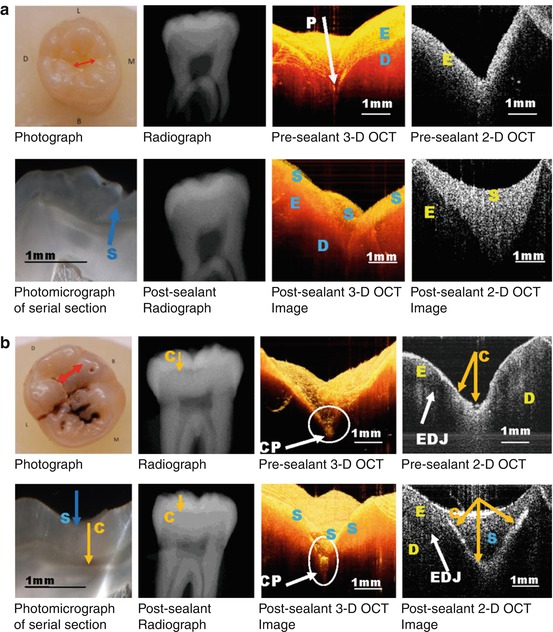
Fig. 74.11
Typical pre- and post-sealant photographic, radiographic, and OCT images as well as photomicrograph of histological section of healthy tooth (a) and carious tooth (b). (a) Images of healthy tooth sample pre- and post-sealant (Fuji TRIAGE sealant) Above: LHS: photograph with red arrow indicating OCT scan line; Center left: pre-sealant radiograph; Center right: pre-sealant 3D OCT image; RHS: pre-sealant 2D OCT image. Below: LHS: photomicrograph of histological section of tooth, blue arrow indicates sealant; Center left: post-sealant radiograph; Center right: post-sealant 3D OCT image; RHS: post-sealant 2D OCT image. P-occlusal pit; E enamel, D dentin, S sealant. (b) Images of decayed tooth sample pre- and post-sealant (Delton sealant) Above: LHS: photograph with red arrow indicating OCT scan line; Center left: pre-sealant radiograph with yellow arrow indicating caries (C); Center right: pre-sealant 3D OCT image with carious occlusal pit (CP) indicated by white arrow; RHS: pre-sealant 2D OCT image with occlusal caries (C) indicated by yellow arrow and EDJ indicated by white arrow. Below: LHS: photomicrograph of histological section of tooth, blue arrow indicates sealant (S), and yellow arrow indicates caries; Center left: post-sealant radiograph with yellow arrow indicating caries (C); Center right: post-sealant 3D OCT image with carious occlusal pit (CP) indicated by white arrow; RHS: post-sealant 2D OCT image where white arrow indicates EDJ and yellow arrow indicates caries. P-occlusal pit; E enamel, D dentin; S sealant, C caries, CP carious pit, EDJ enamel-dentin junction [58]
A paper by Jones et al. described the effective use of polarization-sensitive optical coherence tomography (PS-OCT) to image lesions underneath composite sealants [59]. The polarization-sensitive system, recording images in both the parallel and perpendicular axes, was useful for enhancing the image contrast of the artificial caries and minimizing the interference of the strong reflections at surface interfaces. The artificial lesions could be detected under 750 μm of visibly opaque sealant. Tooth-colored sealants allow deeper imaging depth. The artificial lesions could be detected under 1,000 μm of sealant.
Finally, OCT can be used to assess the internal structure and marginal adaptation of cemented crowns. Cross-sectional images were made in duplicate on the midfacial surface of cemented functional crowns with well-adapted margins and no clinical evidence of recurrent caries. The internal structure and marginal adaptation of porcelain fused to metal crowns were clearly visualized in teeth imaged in vivo. Characteristic image layers that corresponded to incisal and body porcelain were visualized [60].
Using three-dimensional OCT imaging, it is possible to evaluate the structural integrity and wear of composite resin restorations. An OCT contour map of restoration surfaces can be used to quantify volumetric loss or surface changes in a restorative material over time. Wear estimates correlate well with the value as determined by the traditional method of measuring composite wear, the ML scale (r = 0.86; p < 0.05). OCT images can record changes in the restoration surface that occurred with occlusal function. It was also shown that a region of catastrophic failure not visible clinically or radiographically was detected by OCT [61].
74.2 Periodontal Tissues
Periodontal diseases are a major cause of tooth loss. They are plaque-induced inflammatory disorders that result in the loss of tooth support through the apical migration of connective tissue attachment which results in the resorption of alveolar bone. To establish the diagnosis of periodontitis, it is necessary to detect the loss of connective tissue attachment and alveolar bone [62]. Traditionally, the location of the periodontal attachment has been estimated by mechanically measuring the distance a probe penetrates the soft tissues surrounding the tooth. Attachment level is defined clinically as the distance from a fixed reference point on the tooth (the cementoenamel junction) to the most apical extent of probe penetration. Probing depth is the distance from the gingival margin to the most apical extent of probe penetration. Accurate assessment of probing depth and changes in soft tissue attachment level are critically important in periodontics.
Current methods of periodontal probing use conventional, mechanical, or pressure-sensitive probes. Unfortunately, as a measurement technique, periodontal probing has several sources of error that make it somewhat imprecise [63]. For example, the extent of probe penetration varies with insertion force [64], inflammatory status of the tissues [65], and diameter of the probe tip [66, 67]. Therefore, under routine clinical conditions, periodontal probes have an average measurement error of approximately ± 1 mm [68]. Examiner variation is a major source of error for conventional probes. Training and calibrating examiners decrease but do not eliminate examiner error. Probing errors also result from patient characteristics, such as anatomical differences in tooth contour, particularly in furcation areas. Probing error varies for different sites within the mouth [69]. Patient discomfort can also result in erroneous measurements [69]. Measurement error limits the ability to identify subtle differences in clinical values, which may be important in early disease detection and intervention. These errors diminish the likelihood of accurately identifying etiological factors correlated with disease progression. Finally, a fundamental limitation is that probing cannot identify disease activity status – which is important, given the cyclical and intermittent nature of periodontal disease activity.
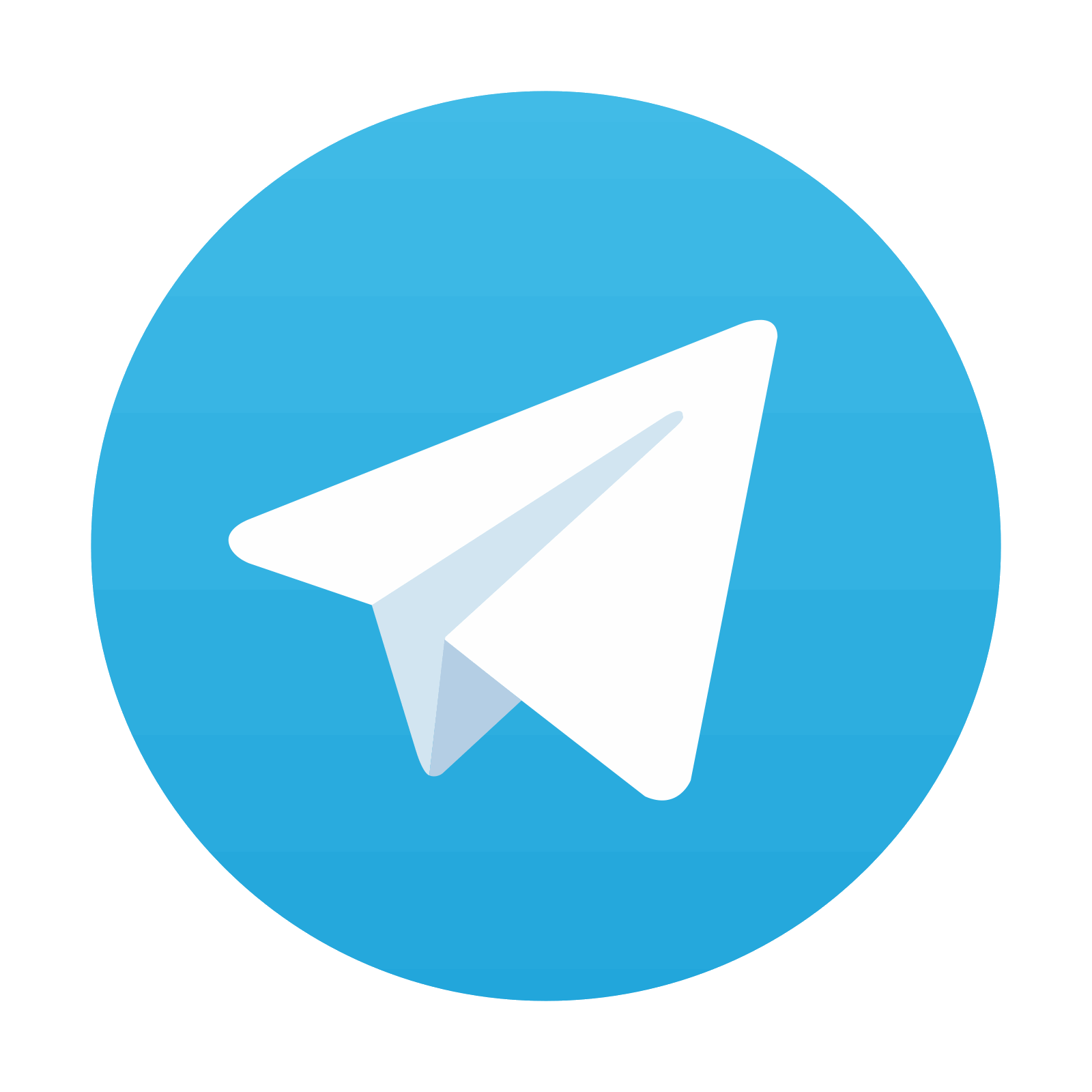
Stay updated, free articles. Join our Telegram channel
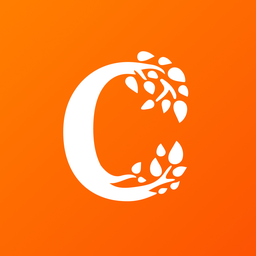
Full access? Get Clinical Tree
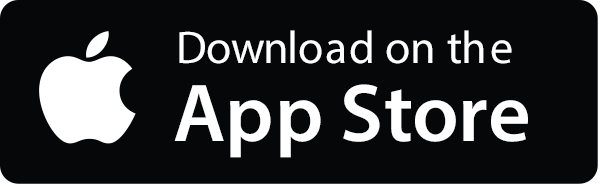
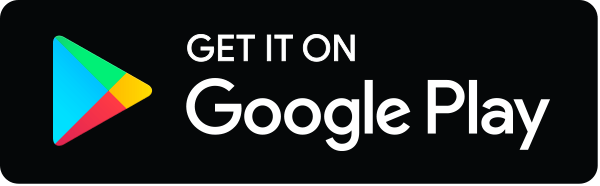