Fig. 10.1
Zinc Finger Nucleases introduce site-specific DNA double strand break and the damaged gene is replaced by homologous recombination containing desired gene and a selective marker
To date, gene therapy has primarily been considered as a treatment for mature, differentiated tissues, with an emphasis on replacing the disease-causing genes directly. But the emergence of human-induced pluripotent (iPS) cells has ushered in a new era of possibilities within gene therapy. iPS cells have advantages that are clear even in their name; they are induced from cell cultures, as opposed to derived from embryonic lines, and they are pluripotent, or can become almost any kind of cell. Capitalizing on this ability, stem cell researchers can modify iPS cells through homologous recombination, differentiate them fully, then transplant them back into the host to treat the disease. Preliminary studies of gene therapy on iPS cells have proven successful in animal models. Such ex-vivo gene therapies, while still in development, could become a possible cure for many hereditary conditions.
This review will begin by describing current ex-vivo gene therapy strategies.
Gene Addition and Replacement: Two Types of Therapies
We propose that the current ex-vivo gene modification therapies can be roughly classified into two categories: the more traditional ex-vivo gene addition therapy and newer ex-vivo gene replacement therapy (also known as gene correction therapy). We propose that the current ex-vivo genemodification therapies can be roughly classified into two categories: the more traditional ex-vivo gene addition therapy and newer ex-vivo gene replacement therapy (also known as gene correction therapy). Both take place primarily outside the body (hence, ex-vivo). To prepare for the insertion of the therapeutic gene, target cells must first be harvested from the patient and maintained in a culture. In gene addition, a viral vector housing a copy of the therapeutic gene is then injected into the cells, where it genetically alters them by the process of transduction. Finally, the genetically modified cells are re-implanted into the patient, where they restore normal functioning of the gene.
A major risk posed by gene addition is the possibility that random portions of the viral genome will become integrated into recipient cells, and that these will make their way into the patient through the transplant or injection procedure. Such random integrations, referred to as insertional mutageneses, would increase the risk of cancer for the patient [2]. The regulation of the therapeutic gene must equally be taken into consideration. The therapeutic gene needs to be expressed by a promoter, a major region of DNA within the gene that facilitates the transcription and expression of the gene. In general, a strong promoter is used in gene therapy to ensure the therapeutic gene is sufficiently expressed. For instance, a constitutive promoter allows the gene to be continuously transcribed without regulation and without regard to its environment. However, this also poses a danger: the constitutive promoter may go overboard in expressing the promoter and might never stop transcribing new copies, an outcome that would be difficult to regulate in vivo. In contrast, intrinsic promoters, which are currently being tested as an alternative means for promoting gene expression, are well regulated; they can prompt a therapeutic gene to produce certain proteins as necessary but also shut down in the normal environment. In terms of regulation, the intrinsic promoter is preferable for traditional gene therapy.
The process of ex-vivo gene correction therapy is similar to that of gene addition therapy, but involves a different mechanism for transferring the therapeutic gene. In gene correction, the abnormal gene is excised and replaced with a therapeutic gene [3]. In comparison, gene replacement localizes the problematic gene and breaks it off using ZFNs or TALENs, creating a space for insertion of the healthy gene. Homologous recombination is used to perform the crucial replacement event. Homologous recombination occurs often throughout the chromosomes of a healthy body; it is the same mechanism used to repair broken or damaged DNA, or to introduce genetic variation from both parents during meiosis. Gene targeting therapies use homologous recombination to align the positions of the therapeutic gene with the patient’s mutant gene and to replace them with one another.
Several selection processes are used to ensure that only successful products of this ex-vivo procedure are actually implanted. Whenever using homologous recombination in gene therapy, an antibiotic-resistance gene is added to the engineered construct region as a positive selection marker. In addition, a negative selection marker, the tk gene, is added to the periphery of the sequence’s similarity region. The cells used for the transplant are grown in antibiotics to select for recombination, and in gancyclovir to kill any cells that carry random integrants. The antibiotic-resistance gene is then removed by a site-specific recombination system such as Cre/LoxP or Flp/FRT [4].
Arguably, this type of gene correction therapy is a safer and less cumbersome approach than gene addition; with gene correction, less genetic material is inserted overall. Moreover, the gene addition approach requires a mechanism by which the therapeutic gene can be expressed; an expression cassette containing a promoter must be inserted into the patient’s genome, where it can activate the therapeutic gene. Gene correction only requires inserting the therapeutic gene and typically 34 additional base pairs.
Site-Directed Engineering of Replacement Genes in Stem Cells
Researchers have long been fascinated by the possibility of applying site-directed engineering to stem cells. Homologous recombination techniques prove most useful for these applications, since they allow for the targeted engineering of replacement genes in the stem cells. In 1989, Schwartzberg et al. reported creating germline modifications in mouse stem cells, changes to the mouse’s genome that would carry on to future generations, using homologous recombination-based genetic engineering [5]. In 1989, Mario Capecci won the Nobel Prize for a discovery making use of this method: the so-called “knockout mouse,” valuable for researchers because of the opportunities it presents to study the effects of having a “knocked out” or absent version of a particular gene within an animal model. Knockout mice typically differ from wild-type mice by just one “knocked-out” gene; site-specific engineering makes such specificity possible.
The procedure for preparing knockout mice is a prime example of targeted gene therapy in action. First, embryonic stem cells are derived from non-genetically engineered mice. These stem cells are kept alive and cultured in a petri dish. The genetic area to be modified must be closely isolated and characterized during this period via the generation of subclones. An appropriate construct must be created, containing a selectable marker: for instance, antibiotic-resistance genes. When the cells are sufficiently cultured they are transfected with the construct. The cells must then be grown in a medium with antibiotics, allowing for the elimination of unsuccessful cells and the identification of successful ones. The successful cells express the desired gene.
These cells are then injected into blastocysts, embryos containing target clones, after which the blastocysts are transferred into pseudopregnant female mice (mice that are not pregnant but have hormonal levels that mimic the levels found in pregnancy). The result is the first generation of filial 0 (F0) chimeric mice that contain the modified genes. Chimeric mice are mated with unaltered mice, resulting in a filial 1 (F1) germline transmission of modified embryonic stem cells. These are the gene knockout mice that serve as the disease model [6, 7].
Of the species currently used in targeted gene therapy research, mice are the most similar to humans. Of late, homologous recombination methods have proven to be a powerful tool for elucidating gene function and have yielded insight into animal models of disease. The effects of lacking a particular gene on the phenotype of the mouse can help elucidate the effects of disease on human subjects. These can be used to test new treatments and drugs. For instance, in 1996, Chang et al. developed a mouse model featuring the inactivation of the alpha-globin gene using gene targeting to disrupt the 5′ alpha-globin gene of the mouse [8]. Since 1996, this model has been available for studies in hematology research. More recently, the p53 gene knockout mouse model has yielded information about perhaps the best known genetic lesion leading to human cancer, the mutation of the p53 tumor suppressor [9]. The use of knockout mice in ophthalmology research has become widespread.
While the mouse models created via homologous recombination and embryonic stem cells have proven extremely useful for drawing inferences about human diseases and treatment effects, there are still limits to the conclusiveness of mouse model studies. The cellular and physiological differences between mice and humans are too great to assume that genes are expressed similarly in mice and humans.
It is now becoming more feasible to create more targeted mouse models using homologous recombination to reengineer human embryonic stem cells (hESCs). Embryonic stem cell lines are self-renewable, with a high pluripotency that allows them to differentiate into almost all cell types [10]. Recently, homologous recombination techniques that use standard plasmid-based vectors to target endogenous genes have been tested on hESCs to see whether it is possible to modify them [11–17]. However, these procedures remain challenging in embryonic stem cells. To date, there have been few successful occurrences of homozygous disruption of a gene in hESCs. In addition, the natural rate of homologous recombination in genes is low, around one in 1 × 105 to 1 × 108 [18, 19], well below the standard for therapeutic use. It is necessary to raise the efficiency of the recombination event.
Song et al. have proposed one possible solution: the use of bacterial artificial chromosomes, vectors with large homologous arms that have been used to clone large sequences of DNA [20]. A recent study found that a BAC-based targeting vector was highly successful in disrupting targeted genes in hESCs.
Nonetheless, the use of artificial chromosomes poses a new challenge: it is difficult to confirm the homologous recombination event has taken place. Other recent studies have shown that the use of engineered enzymes to create a site-specific DNA double-strand break enables a high homologous recombination rate.
Zinc Finger Nucleases Increase Rates of Homologous Recombination in Gene Therapy
Zinc finger nucleases (ZFNs) are artificially engineered restriction enzymes created by fusing a zinc finger DNA-binding domain made up of Cy2His2 to a nonspecific DNA-cleavage domain, typically the type IIS endonuclease Fokl [19]. The ZFNs’ fusion proteins have been reported to stimulate localized mutagenesis and/or homologous recombination, while their DNA-binding domains provide high binding specificity to particular DNA sequences.
ZFNs contain two arrays, left and right, each consisting of three zinc fingers. Each zinc finger makes contact with three base pairs; each of the two arrays makes contact with about nine base pairs of the DNA sequence in total [19]. These fingers can be ordered in a multitude of combinations. Between the two arrays is a spacer region consisting of 5–7 base pairs, a cleavage domain derived from the type II restriction endonuclease Fokl. This section of the zinc finger nuclease dimerizes and allows the ZFNs to bind to the target sequence. The FokI cleavage domain can recognize a target sequence of DNA of up to 23–25 base pairs in length and break each end of it, creating a double-strand break. These characteristics make ZFNs useful tools in homologous recombination.
The precise genome modifications created by ZFNs fall under two categories. A ZFN-induced double-strand break can result in non-homologous end-joining, causing small mutations such as insertions or deletions. Although these mutations induced by ZFNs are not controlled, this technique is still used to create knockout cell lines in a variety of organisms. For example, in human disease research, non-homologous ZNF-induced breaks have already been used to add targeted mutations to human T cell CCR5 genes, yielding T cells that are resistant to human immunodeficiency virus (HIV) infection [21]. This research has entered phase I clinical trials.
Secondly, ZFNs can modify the genome by creating site-specific DNA double-strand breaks and repairing these breaks via homologous recombination. In 2007, Lombardo et al. developed a technique for performing homologous recombination on hESCs using ZFNs [22]. Theoretically, this technique allows for making either small changes (on the order of a single nucleotide) or large ones (on the order of a large transgene cassette). Recent studies further demonstrated that the site-specific DNA double-strand break induced by ZFNs stimulates the homologous recombination process up to several 1,000-fold [23–27]. ZFN-induced homologous recombination, with its relatively high efficiency, is thus a promising tool for researchers, one with high therapeutic value for treating human disease [28–30].
However, even though ZFNs have demonstrated a high specificity when inducing double-strand breaks and have been shown to stimulate homologous recombination in vivo, their widespread adoption is still hindered by the lack of a robust, publicly available database for engineering zinc-finger arrays. The three zinc fingers of the ZFN can consist of a large variety of combinations that impact its binding specificity. A “modular assembly” approach could be used to join zinc finger modules into arrays. The procedures for accomplishing this are technically simple, but currently still inefficient, with a high rate of failure by trial and error. A formal database or library of ZFN, if made widely available, would present a solution to this problem.
In the past, ZFN creation methods have made use of oligomerized pool engineering (OPEN), an open-source, combinatorial sequencing-based protocol for building zinc fingers. OPEN utilizes the databases of the zinc finger pool, which determines the three base pair subsite of each zinc finger and randomly recombines them to form a random zing finger array library. However, the process of building and screening a combinatorial ZFN library is time consuming and labor intensive, limiting the broader adoption of this method [31].
The company Sangamo Bioscience has developed a platform for making four-zinc finger ZFNs. Although some detailed information about this method is already in publication, researchers must gain access to the proprietary database in order to be able to implement the ZFNs. The ZFNs developed from Sangamo Bioscience can be purchased from Sigma-Aldrich under the brand name of CompoZ. However, the limited accessibility of this source has limited its scope and scale.
A final method for generating ZFNs is provided by the context-dependent assembly (CoDA) platform. With this approach, two three-zinc finger arrays determined to contain a common middle zinc finger are assembled using N- and C- terminal fingers to form customized zinc finger arrays. The first finger is taken from one zinc finger array and the third finger is taken from the other. Nonetheless, this CoDA method constrains the identity of the middle zinc finger. It also leaves unchecked the effects of the three zinc fingers on the affinity and the specificity of the final zinc finger array. For these reasons, this method is less preferable, especially in highly demanding therapeutic settings [32].
Overall ZFNs have been proven to have a high targeting specificity and many applications. However, the widespread use of ZFN-based gene therapy has been limited by multiple factors; namely, the high cost and the labor intensiveness of generating ZFNs.
Transcription Activator-Like Effector Nucleases (TALENs) Can Induce Site-Specific Double Strand Breaks
Induced double-strand breaks have a proven ability to disrupt gene sequences by two means: through non-homologous end-joining repair systems, or through homologous recombination repair pathways, with an exogenous plasmid as template. Like ZFNs, transcription activator-like effector nucleases, or TALENs, have been proven to induce double-strand breaks. TALENs are engineered DNA binding proteins which fuse transcription activator-like (TAL) effectors to a DNA binding domain. TALENs can recognize specific DNA sequences.
TAL effectors are produced by the Xanthomonas genus of plant pathogens. They activate transcription by binding to the effector sequence on the host cell promoter, once delivered into the host cells through type III secretion pathways [33]. The DNA binding domain of TAL effectors consists of tandem 33–35 amino acid repeat modules followed by truncated repeats of 20 amino acids. Among the amino acids in the DNA binding domain, the adjacent 12th and 13th amino acids are highly variable, while the remaining residues are nearly identical in each unit. These two high-variable amino acids, called repeat variable di-residues (RVD), are in charge of specifying the DNA binding target. Changing the RVDs makes the TAL effectors target different nucleotides with a high level of specificity.
Not only is the simple, straightforward sequence structuring convenient for predicting TAL effectors’ DNA binding sites, but it also allows for the construction of custom TAL effectors [34, 35]. These engineered TAL effectors are proteins that fuse the TAL effectors with the catalytic domain of FokI nuclease: these are the transcription activator-like effector nucleases or TALENs. The FokI catalytic domain functions within a pair of dimers to create the double-strand break at the specific target sequence.
To generate customized TALENs to target specific sequences, previous research has suggested that the customized amino acid repeats of the DNA binding domain could be constructed through the sequential cloning of sequence-verified single, double, and triple repeat modules [36]. With this method, array integrity of TALENs’ DNA binding domain repeats can be assured. Nonetheless, the proposed process is time consuming and labor intensive. Another alternative is to utilize methods based on a polymerase chain reaction (PCR) to generate sequences of amino acid repeats in the DNA binding domain. This method would provide a faster and easier process for TALEN generation, but would also create a risk of sequence mutation or recombined repeats. Overall, the recently developed Golden Gate cloning technique has been reported to be the most efficient methodology for assembling multiple DNA fragment in an ordered fashion in one single reaction to generate customized TALENs [37].
Despite the relative newness of methods for using TALENs in gene targeting compared with the ZFNs, they provide many advantages. One study reports that the mutagenesis frequency of TALENs in transfected cells is estimated to be equivalent to or even 25 % higher than the rate in ZFNs [37]. Because of the simple structure of the TALENs, it is also easier to predict and manufacture customized TALENs to provide a high-targeting capacity. Moreover, TALENs have the ability to target some genes that have been reported as being particularly difficult to target with ZFNs.
Several studies have examined these unique properties of TALENs. In one study, Maeder and colleagues found dramatic differences in ZFNs’ and TALENs’ ability to locate and cleave to a three point deletion mutation associated with a cystic fibrosis transmembrane conductance regulator (CTFR). ZFNs were found to remain at least 120 base pairs away from the target site. Meanwhile, the target sequence resided well within TALENs’ cleavage domain without compromising gene targeting efficiency [46]. Another study on targeting of the acetolactate synthase gene to create herbicide resistant tobacco plants found ZFNs at least 188 base pairs away from the desired side. The same study showed that TALENs cleaving to the site within 10 base pairs of the target sequence. Lastly, ZFNs have notable difficulties targeting the AT-rich regions in DNA. The same study showed that TALENs can successfully target two sites in the genome that contain 80.6 % AT [37].
These outstanding research results, combined with the TALENs’ unique structural simplicity and manipulability make them not only an alternative of ZFNs for procedures inducing a site-specific DNA double-strand break, but also a remarkable tool for genome engineering on the whole. Nonetheless, issues such as TALENs’ cleavage domain array length and need to customize high-affinity RVD arrays still remain to be addressed.
Viral Vectors Currently Used for Gene Delivery
Many different therapeutic gene delivering vectors have been developed since the beginning of gene therapy research in 1970s, including adenovirus vectors, lentivirus vectors, and herpes virus vectors.
Adenoviral Vector
Adenoviruses were first discovered in 1953. They are non-enveloped viruses; they consist of a protein core that contains a linear, double-strand DNA genome of 36–38 kb, and a large surrounding icosahedral protein shell, 70–90 nm in diameter. The protein shell is made up of 252 structures known as capsomeres: hexons, pentons, and fibers. The 12 pentons occupy the vertical part of the icosahedron. A slender projection called a fiber emerges from the base of each penton. The icosahedron has twenty faces made up of 240 capsomeres; these are called hexons because they form hexagonal arrays [38].
< div class='tao-gold-member'>
Only gold members can continue reading. Log In or Register a > to continue
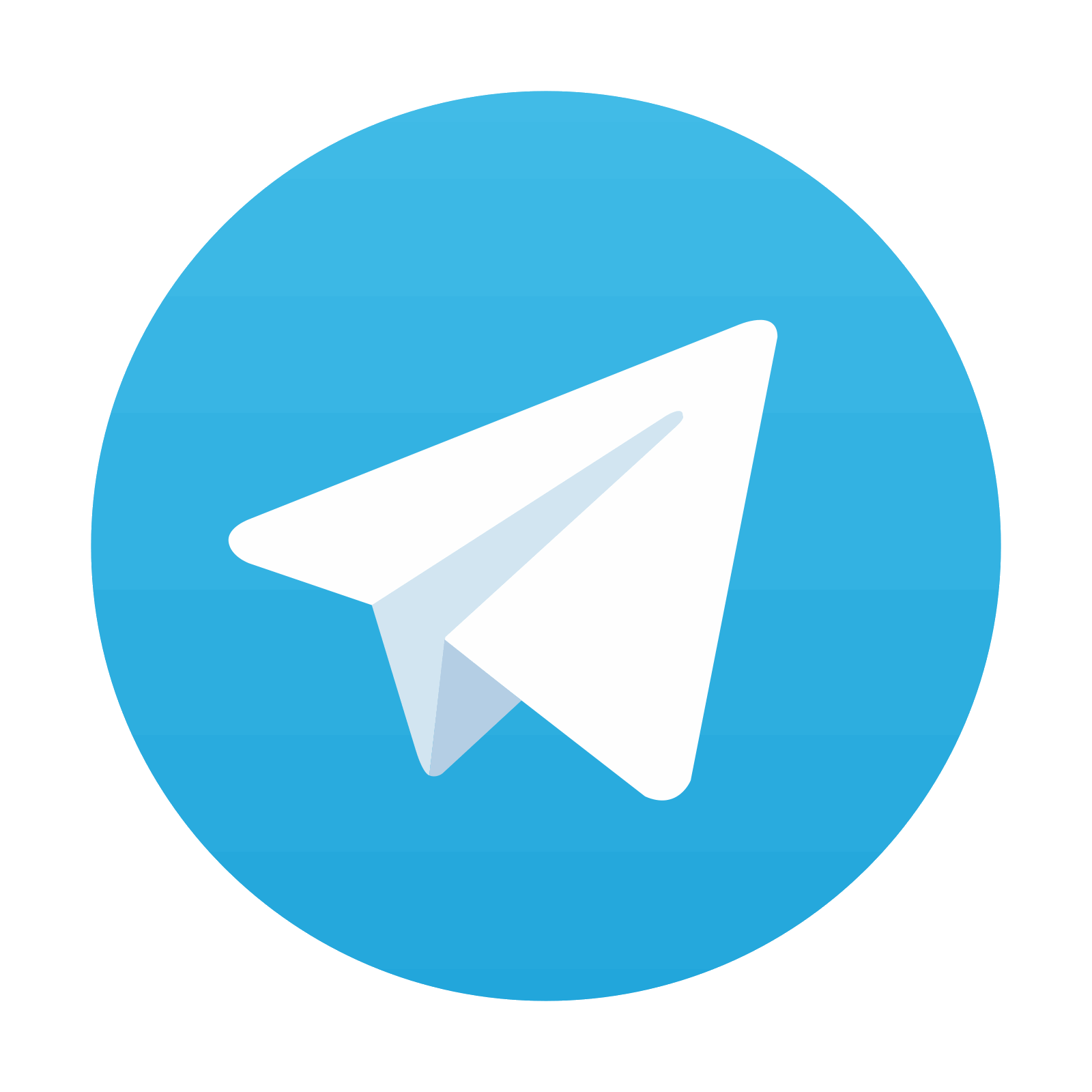
Stay updated, free articles. Join our Telegram channel
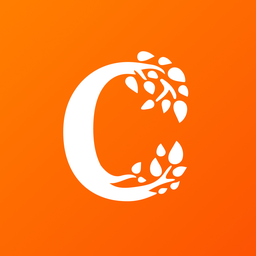
Full access? Get Clinical Tree
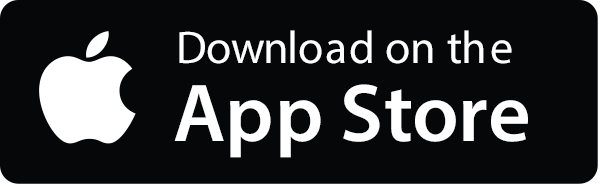
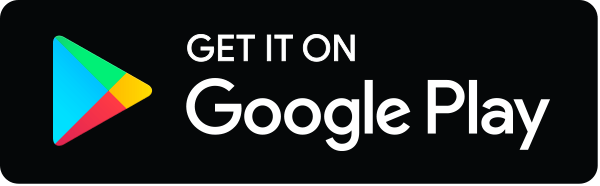