Organ site
# Subjects/samples
Sensitivity
Specificity
Source (year, reference, organization, ex wavelength/notes)
Cervix (colposcopy)
Meta-analysis
Many
Many
2007, [173] MDACC
1,090
56 %
87 %
2007, [174] Medispectra, 337
604/1,500
92 % (67 %)
50 % (54 %)
2004, [68] Medispectra, 337, mc, hg
147/351
71 %
77 %
2002, [70] UT, multi ex, hg
44/84
92 %
71 %
2002, [71] MIT, trim, SIL-Bx. nonSIL
95/381
79 % (79 %)
78 % (76 %)
1996, [175] UT, 337, 380, 460, hg
Ovary
49/249
88 %
93 %
2012, [87] UA, 270–550
30
100 %
69 %
2012, [176] UA, 365, imaging
4
N.A.
N.A.
2011, [112] Groningen, Folate-FITC, intrao
22/97
100 %
91 %
2009, [177] Manipal, 325
Fallopian tube
47
73 %
83 %
2011, [88] BCCA, imaging
Lung (wl bronchoscopy)
62
91 % (51 %)
26 % (50 %)
2006, [81] Hong Kong, 380–460
1,173/1,978
82.3 % (57.9 %)
58.4 % (62 %)
2005, [78] Storz, mc, hg
32/62
80 %
83 %
2004, [80] Olympus
173/864
67 % (25 %)
66 % (90 %)
1998, [77] Xillix, mc, hg
Oral cavity (wl examination)
120
N.A.
N.A.
2009/10, [178, 179] Houston, 405, vis
44/50
98 %
100 %
2009, [180] BCCA, 400–460, hg, vis
4/4 (images)
91 % (75 %)
86 % (43 %)
2005, [181, 182] UT, visual
15/91
96 %
96 %
2003, [98] MIT, trim
76/343
100 % (100 %)
88 % (83 %)
2002, [183] UT, multi ex
56/179
100 %
51 %
2002, [184] Munich, ALA,375–440
50/137
88 %
57 %
AF
7/199 (72)/(214)
100 % (99 %)
51 % (43 %)
ALA&AF (WL)
15/45
94 % (76.5 %)
100 % (100 %)
1998, [185] UT,MDACC, 337, 365, 410
Breast
6
N.A.
N.A.
2009, [115] ICG, lymph node intrao
N.A.
N.A.
N.A.
2009, [186] Manipal, 325
17/104
100 %
96 %
2008, [187] MIT, multi ex
3
N.A.
N.A.
2007, [99] Storz, Ductoscopy
18/47
54 %
91 %
2005, [188] UW, multi ex
32/56
70 %
92 %
2003, [101, 116] UW, multi ex
63/911
99 %
99 %
1997, [189], Indore, 337
Colon (wl colonoscopy)
21
N.A.
N.A.
2013, [96] UA, 270–440
88
Better than WL
N.A.
2012, [92] Asahikawa, AFI
75
92 % (68 %)
N.A.
2001/10, [90, 190] PINPOINT, 400–450
64
84 % (90 %)
60 % (64 %)
2007/8, [89, 191] AFI, 395–475
NA/177
80 %
92 %
1992, [192] Wellman, 337
Barrett’s esophagus
22
100 %
Increase
2012, [102], Amsterdam, AFI, NBI, HRE
37/148
74 %
85 %
2003, [103] Wellman, 400
16
100 %
97 %
2001, [104], MIT, 337, 397, 412, trim
53/141
76 %
63 %
2003, [193] Lausanne, 505
Bladder
14/56
N.A.
N.A.
2013, [109] 308
21
N.A.
N.A.
2012, [108], 650
25/52
100 %
100 %
2003, [106] Singapore, 280, 330
25/43
95/84 %
42/88 %
2001, [107] Munich, AFI alone, ALA & AFI
75/130
95 %
73 %
1998, [105] Wellman, 337
Brain
3/13
N.A.
N.A.
2010, [110] UCDavis, 337 lifetime
26/120
100 %
76 %
2001, [194] VU, primary tumor
97 %
95 %
2004, [195] data from [194]
1.
Cervix: Accurate optical diagnosis of cervical dysplasia could lead to a method where patients with an abnormal cytological screening result are triaged into those who do not need any further intervention and those who need a biopsy or potentially a treatment in the same visit. Several groups have reported recent results including a multicenter clinical trials by MediSpectra [68] with a single wavelength excitation source combined with diffuse reflectance measurements and a point-scanning device as well as SpectRx guided with similar approach [69]. The MediSpectra report indicated a 33 % increase in detection rate for high-grade disease while maintaining the specificity at the level of current clinical practice, while SpectRx also reported reduction in false-positive rate. In 2002 Chang and coworkers [70] as well as Georgakoudi and coworkers [71] published results of their studies on 147 and 44 patients, respectively. Chang reported that more than three to four excitation wavelengths do not improve performance and confirmed previous findings by Ramanujam [72]. He also reported low performance in detecting low-grade disease and distinguishing normal columnar tissue from high-grade disease. Georgakoudi reported results using an analytical model for extracting intrinsic fluorescence [73], reduced scattering and absorption [74], as well as estimates for average number and size of main scatterers [75]. Sensitivity was significantly improved when a combined trimodal approach was used while the specificity was maintained.
2.
Lung: The LIFE systems from Xillix uses excitation between 380 and 460 nm emission and observes fluorescence in the green and red [76, 77]. The D-Light system® from Storz incorporates an additional white-light measurements [78], and the AFI system from Olympus utilizes two additional reflectance channels at 550 and 610 nm [79, 80]. The Pentax bronchoscope SAFE-1000 used blue excitation and observed green autofluorescence [81]. A European multicenter trial with the D-Light system® reported increased sensitivity [78] compared with the earlier multicenter trial by Lam et al. [76] with the Xillix system and corrected the sensitivity and specificity of white-light bronchoscopy reported by Lam. The AFI system was tested on a small sample size, and only abnormal-appearing sites were included in the analysis [80]. A relatively high false-positive rate was reported which limits the positive predictive value [82], and difficulties in distinguishing preinvasive lesions and benign conditions were mentioned. However, a trial with the Pentax system also reported increased sensitivity but reduced specificity [81].
3.
Skin: A skin autofluorescence reader has been developed to asses advanced glycation end products (AGEs) which show a fair correlation with collagen cross-link and AGE content of the skin (AGE Reader I, DiagnOptics BV Groningen, Netherlands) [83]. Such reading could be a predictor of health outcomes in diabetic patients [84] or could determine cardiovascular risk [85, 86].
4.
Ovary: Tissue fluorescence was found to be statistically different in normal ovarian tissue between women at high risk for developing ovarian cancer versus women at normal risk especially in postmenopausal women; this result can potentially guide oophorectomy [87]. With the recent hypothesis that ovarian cancer might originate in the fallopian tube, it has been demonstrated that autofluorescence can be measured in the fallopian tubes in situ [88].
5.
Colon: Xillix and Olympus developed autofluorescence (AF) colonoscopes that illuminate tissue in the violet–blue range. Several randomized trials comparing AF endoscopy in the colon to standard video endoscopy, narrowband imaging (NBI), or high-resolution endoscopy have been published very recently. The outcomes of these studies have been mixed, with some indicating these AF endoscopes reduce polyp miss rate [89–92], especially for inexperienced colposcopists, and others showing no significant improvement of AF over other technologies [93–95]. Most recently UV imaging has been proposed to improve the detection rate of polyps [96, 97].
6.
Oral Cavity: Two companies have created a visual autofluorescence system for the examination of the oral cavity. The VelScope™ (LED Medical) and Identafi™ (Remicalm) illuminate the tissue with specific excitation pattern, while the clinician observes the tissue fluorescence through filtered spectacles. The VelScope™ uses blue (400–460 nm) excitation light, while the Identafi™ uses light at 405 nm in combination with green reflectance. The devices have been identified as ideal for oral cancer screening but also for tumor margin delineation. Clinical performance is supported by previous studies using single point spectroscopy and multiple excitation wavelengths [98].
7.
Breast: While there were limited reports of clinical translation of LIF for breast cancer imaging, there exists a commercial implementation of 1.3 mm diameter ductoscope with autofluorescence imaging capabilities using the DAFE system (Richard Wolf GmbH) [99]. Previously research with fiber-optic probes reported that diffuse reflectance spectroscopy did not improve sensitivity and specificity of fluorescence spectroscopy [100, 101].
8.
Esophagus: Esophageal cancer detection was investigated with technologies similar to the one used for colonoscopy [102], and autofluorescence imaging combined with high-resolution endoscopy was found to have ideal sensitivity. In addition when combined with narrowband reflectance imaging, a reduced false-positive rate was demonstrated. Earlier work showed that single point spectroscopy at 400 nm excitation was most useful for clinical application [103], while others demonstrated excellent diagnostic performance using fluorescence at 337, 397, and 412 nm excitation [104].
9.
10.
11.
Exogenous Contrast Agents: Significant advances in intraoperative optical imaging of exogenous contrast agents have been reported recently. For example, folate-targeted fluorescein isothiocyanate (FITC) fluorescence was used to guide tumor debulking in ovarian cancer patients [112]; patency of bypass grafts were studied in patients using intravascular ICG [113]; and sentinel lymph node mapping was demonstrated in the esophagus in animals [114] and also applied in human studies for breast cancer lymph node mapping using open architecture equipment [115]. General lymphatic imaging was demonstrated using ICG [116]. Aminolevulinic acid (ALA)-induced protoporphyrin IX fluorescence has been studied in several animal models. Its application was studied for peritoneal micrometastasis of ovarian cancer in a rodent animal model and showed that detection rate significantly increased, and fluorescence intensity in tumor versus surrounding tissue increases by 1.5 [117]. Such an approach could be combined with endoscopic devices such as the D-Light system® (Karl Storz, Germany); however, human clinical studies have not yet been reported.
50.4 Advantages of a Dual Modality System
The complementary nature of information provided by OCT and LIF (coherent backscatter versus incoherent fluorescence emission) creates the possibility that the combination of OCT and LIF may be more sensitive to tissue function and pathology than either modality alone. The two types of information may also facilitate signal interpretation and increase specificity. Viewing this potential advantage from two different perspectives, cross-sectional OCT images may help to correct and interpret LIF spectra, or LIF imaging/spectra may help to guide and interpret OCT images.
From the first perspective, LIF spectra may be difficult to interpret because they may be influenced by unknown subsurface structures. Spectra obtained at different lateral locations on an apparently smooth, homogeneous surface can vary in intensity and spectral shape. Without depth-resolved anatomical information, it may be difficult to determine if the variation is due to changes in tissue function, type, or thickness. For example, in a layered epithelial/stromal tissue, a relative decrease in the intensity of the fluorescence signal associated with collagen could be due to a true lessening of the fluorescence emission of the stroma or could be caused by a thickening of the overlying epithelium. Another example is given in Fig. 50.1. An OCT image/LIF spectra pair is shown, obtained from the luminal surface of an approximately 3 mm diameter bronchus of excised sheep lung. The spectral shape of the LIF signal is relatively constant, but the intensity is highly nonuniform. The OCT image shows clearly that strong LIF signal corresponds to locations with subsurface cartilage, indicating that the LIF intensity variation is due to normal anatomy.
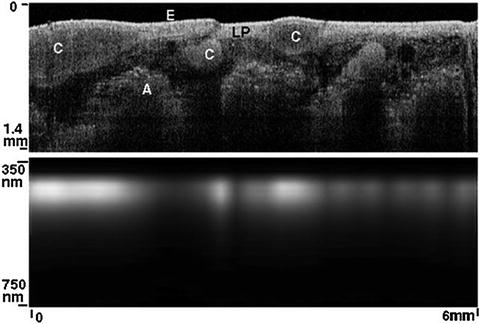
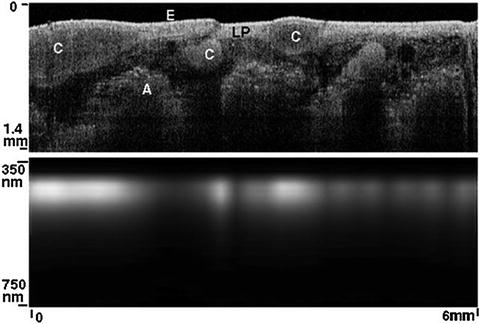
Fig. 50.1
OCT image (top) and LIF spectra (bottom) of excised sheep lung bronchus. Note the increase in emission intensity at lateral locations corresponding to subsurface cartilage (C). Other abbreviations: epithelium (E), lamina propria (LP), alveoli (A)
Quantitative measures of tissue anatomy obtained with OCT can be used to correct LIF data. Several attempts have been made to separate tissue optical properties, such as scattering, absorption, and intrinsic fluorescence from extrinsic fluorescence, and to calibrate fluorescence measurements for illumination and detection conditions. The collected fluorescence depends on optical transport within the tissue [82, 118] and geometrical sensor sample relations [119–121]. LIF intensity is highly dependent upon the probe–sample separation, and OCT can provide this geometrical information with high accuracy. In fact, if only point LIF measurements are being obtained, only A-scan OCT capability is needed. A correction algorithm has been described by Warren et al. [119] using ultrasound A-mode data. A model of the fluorescence intensity was developed and verified with both Monte Carlo simulations and empirical data. A correction equation goes as Sc = Sm/Rn, where Sm is the measured fluorescence intensity, R is the probe–tissue separation, and n = 1.1 for aorta and 2 for theoretical, isotropic fluorescence emission.
Using mathematical models, the particular photon traveling paths can be predicted with a priori knowledge of the illumination and collection geometry, a diffuse reflection measurement, and assumptions about scattering and absorption properties. Zhang and coworkers developed a method that allows extraction of intrinsic fluorescence in semi-infinite media based on fluorescence and diffuse reflectance measurements under the same geometrical configuration [122], which subsequently has been used in several clinical studies as an analytical model. Sung et al. developed a similar analytical approach for two-layered tissue to predict intrinsic fluorescence from the stroma and epithelium separately [123]. This model depends on the epithelial thickness, a number that can be easily obtained with OCT. Fluorophore concentration can also be more accurately determined using tissue scattering and fluorophore depth measurements obtained from OCT. Yuan et al. corrected the measured concentration of a known fluorophore (Cy5.5 dye) in a phantom (tube of dye submerged in intralipid) [124]. This method could be employed to help quantity accumulation of, e.g., an exogenous dye in a discrete tumor. It would be less useful for correcting measurements of distributed endogenous fluorophores, although it could be modified to estimate true contributions from a buried layer (e.g., collagenous stroma) if optical properties and fluorophore concentration of tissue layers are assumed to be homogeneous.
From the second perspective, LIF imaging can guide OCT measurements and clarify inconclusive OCT scans. OCT provides a microscopic view, and thus in many applications complete screening of the area of interest is impractical, necessitating a guidance technique. LIF imaging, which commonly has high sensitivity but low specificity, can be an ideal method to identify regions of interest for OCT imaging. A simple method of LIF-guided OCT can be implemented by using an OCT daughter probe in the surgical channel of a large endoscope. Additionally, LIF has the potential to clarify OCT scans containing little information due to homogeneous tissue with features below the resolution limit of OCT, such as might be found in a cellular tumor or scar tissue.
Several studies have shown the value of combining OCT and LIF. Kuranov et al. [125] used OCT and LIF to image neoplasms in the cervix and found that these two modalities combined produced fewer false-positive results than either modality alone: abnormally increased fluorescence due to inflammatory reactions was clearly differentiated from cancer by OCT; conversely, OCT-detected atypical structure could be clarified with LIF to simply be a mature scar. Another study [25] found that the PPV of fluorescence cystoscopy of flat bladder lesions was only 16 %, which could be increased to 43 % in addition of OCT. The data suggest that 78 % of biopsies based on fluorescent-positive findings could be avoided, if areas were classified as normal when OCT imaging revealed a regular layered structure.
50.5 Instrumentation Design Considerations
Combined OCT–LIF systems can be essentially two separate systems simply physically packaged together at the tissue location or can share optical and mechanical parts, light sources, and/or digital processing components. There are many possible variations; here we list some of the design considerations and some current/possible implementations. Design considerations for system combination include the type of OCT and LIF systems desired (e.g., full field or scanning OCT, point or imaging LIF), the spectral range of the light source(s) and LIF emission, the choice of endoscope materials, and safety. General optical design considerations are also discussed.
50.5.1 OCT and LIF Systems
Because various types of OCT systems are discussed in detail elsewhere in this handbook, they will not be discussed here. In general, LIF capability can be added to any type of OCT system, although the sharing of components is facilitated if the scan types are common (e.g., both one, two, or three dimensional scanning and full field). An appropriate method of signal separation (spectral, temporal, or spatial) is required.
Steady-state LIF measurements require a light source with a well-defined output spectrum, a conduit to transport the excitation light to the sample, illumination and collection optics, and a conduit to transport the emitted light back to a spectral analyzer for optical detection. Instrumentation for fluorescence measurements is well described by Lakowicz [39], and here we focus on spectral compatibility and common in vivo measurement limitations.
One of the major challenges in fluorescence detection is the signal to background ratio. Background signals are caused by instrument autofluorescence (see Sect. 50.5.4), ccc from the light source, and scattering within the spectral analyzer. Because endogenous signals can be weak, background signals need to be avoided. Out-of-band illumination suppression should reach 10−5 or 10−6 for endogenous tissue fluorophore measurements, especially in excitation ranges where the fluorescence efficiency is low (blue to green). Because the amount of collected instrument background is also dependent on the sample reflectivity, traditional subtraction of the sample signal by the instrument response to a negative standard (e.g., a measurement in water) has its limitations. As a rule of thumb, instrument background should be kept below 10–30 % of the sample signal. Traditionally, illumination and collection paths have been constructed separately [126] to reduce system autofluorescence. Irradiance levels on excitation optics are significantly higher than on collection optics making it necessary to incorporate autofluorescence reduction techniques in the illumination conduit and optical elements at the endoscope tip. In typical applications, the collection conduit will receive a significant amount of excitation light backscattered by the sample. If the stray light suppression capabilities of the detection system are exceeded (a spectrograph might have 10−4 suppression), the backscattered excitation light can also contribute to the signal background. This can be avoided by dampening excitation light in the detection path with long-pass filters before the emitted light is spectrally analyzed.
50.5.2 Spectral Range of LIF Sources and OCT Sources
For LIF, short-arc lamps have been used as a standard low-cost excitation source when coupled to an excitation monochromator or dielectric band-pass filters. Example radiance data for mercury–xenon and xenon lamps are shown in Fig. 50.2, top panel. Their broad emission range allows wavelength tunability, but a common disadvantage of short-arc lamps is the limited ability to focus light into small optical fibers. A large variety of laser modules has been developed for confocal microscopy, and most of these lasers are compact and sufficiently transportable for endoscopic fluorescence measurements. Example sources are shown in Fig. 50.2 and abbreviated as XeCl, xenon chloride (308 nm emission); HeCd, helium–cadmium (325 nm); N2, nitrogen (337 nm); Ar, argon (351, 364, 458, 488, and 514 nm); YAG, neodymium YAG (1,064, 532 nm); and HeNe, helium–neon (543, 633 nm). Because of the small Stoke’s shift of most fluorophores, narrowband sources are preferred to enable efficient collection efficiency of emission light and rejection of excitation light. In contrast, OCT sources are by necessity broadband, with spectral widths of tens to hundreds of nanometers. Also, OCT sources are generally centered in the NIR, to enable greatest depth of imaging in highly scattering tissue. The light source emission spectra for some OCT sources (short-pulsed lasers, swept-wavelength lasers, and superluminescent diodes) are shown in Fig. 50.2. Also shown in Fig. 50.2 is the extinction spectrum of three tuned nanoshells (exogenous scattering agents) [127].
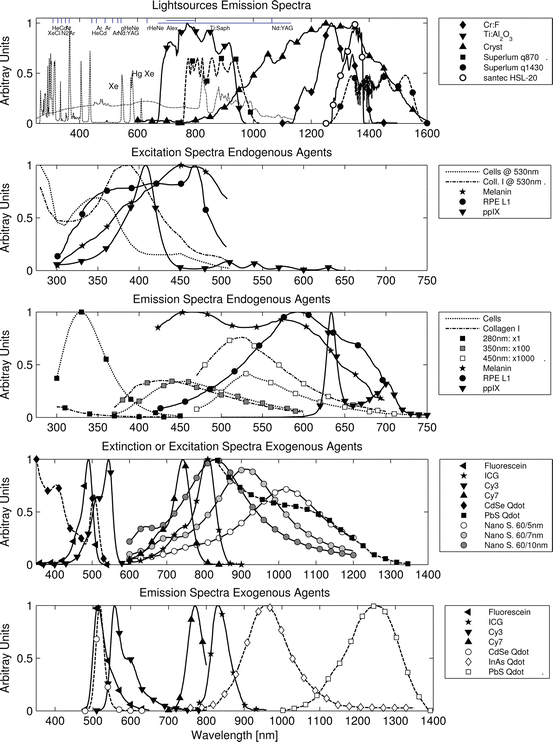
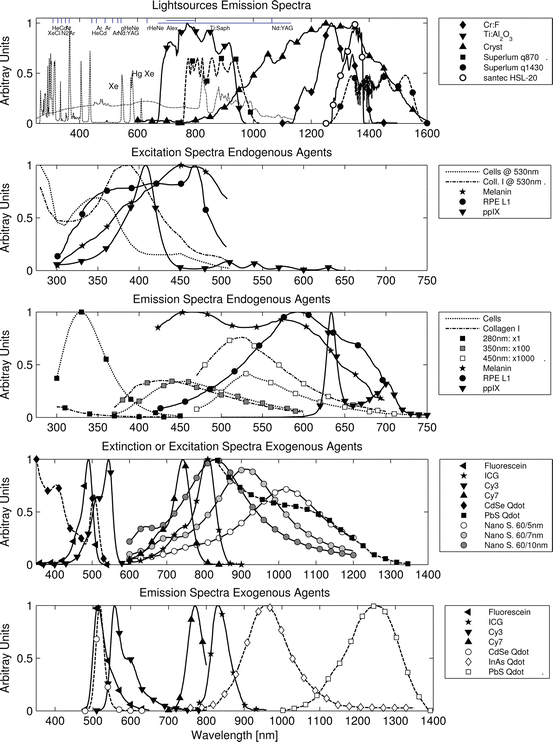
Fig. 50.2
Common light sources, absorbers, and emitters for combined OCT and LIF: First panel, illustration of common fluorescence excitation and OCT light sources. Xenon (Xe) and mercury–xenon (Hg–Xe) short-arc lamps are illustrated (Courtesy of Photon Technology International, Inc.). Published data from OCT sources using titanium sapphire (Ti:Al2O3) laser [196], chromium:forsterite (Cr:F) [197] and photonic crystal fibers (Cryst) [198], commercial superluminescent diodes (Superlum Broadlighters q870 and q1430), as well as spectrum of commercial swept source (santec, HSL-20) are shown. Excitation spectra of endogenous components are illustrated in the second panel: reconstituted collagen type I gel and epithelial cell suspension (OVCA 430) data is shown at 530 nm emission [199, 200]. Protoporphyrin IX [201] and melanin and lipofuscin granules extracted from RPE cells are illustrated [55]. Emission spectra corresponding to the compounds from the second panel are illustrated in the third panel: emission of collagen type I gel and an epithelial cell suspension is shown at 280, 350, and 450 nm excitation. The fourth and fifth panels illustrate excitation and emission spectra of exogenous agents: the absorption and emission of fluorescein (Molecular Probes/Invitrogen, ph9) is compared to a CdSe semiconductor nanocrystal [202, 203]. ICG, a clinically used NIR absorber, is illustrated [204]. Cyanine dyes are illustrated with examples of indocarbocyanine (Cy3) and indotricarbocyanine (Cy7) [201]. Extinction of gold nanoshells on a 60 nm silica core is illustrated as a potential OCT scattering contrast agent [127]. Selected quantum dot emission spectra from CdSe [202, 203], InAs [202], as well as PbS [205] are shown
50.5.3 Excitation and Emission and Range of Fluorophores
Epithelial tissues usually exhibit a strong fluorescence peak at 280 nm excitation and 350 nm emission, which is associated with tryptophan fluorescence (Fig. 50.2, bottom panel, cell emission at 280 nm excitation). Cells excited at UV-A wavelengths show strong fluorescence from NADH, which has a maximum at 350 nm excitation and 450 nm emission (Fig. 50.2, cell emission at 350 nm excitation), whereas the oxidized form NAD is not fluorescent [39]. Conversely, FAD fluoresces at a maximum at 450 nm excitation (blue) and 535 nm emission (Fig. 50.2, cell emission at 450 nm excitation), whereas the reduced form FADH2 fluoresces minimally. Lipofuscin has a very broad excitation and emission spectra. The excitation spectrum is notable in particular because its peak at 470 nm is comfortably above the strong excitation bands of many other materials. Fluorescence from interstitial matrix is dominated by collagen; average fluorescence emission from polymerized collagen that was reconstituted from rat tail type I fragments is shown in Fig. 50.2 middle and bottom panel. Typical emission at 280 nm excitation/300 nm emission (consistent with tyrosine), 320 nm excitation/400 nm emission (consistent with the enzymatic cross-links lysyl hydroxypyridoxyline and hydroxylysyl hydroxypyridoxyline), and 370 nm excitation/450 nm emission (consistent with the nonenzymatic cross-links vesperlysine and crossline) can be observed. Unfortunately, at many excitation wavelengths, these collagen emission spectra overlap with the emission observed from cell suspensions. Porphyrins show an excitation maximum at 410 nm and two emission maxima at 630 and 690 nm, while the peak at 630 nm is substantially more intense (Fig. 50.2, protoporphyrin IX). Emission spectra from exogenous fluorophores fluorescein; cyanine dyes Cy3, Cy7, and ICG; and CdSe/InAs quantum dots are also shown in Fig. 50.2. Some of these agents have NIR excitation and/or emission.
Figure 50.2 illustrates that endogenous fluorescence, targeted fluorescence imaging, and optical coherence tomography can be spectrally incorporated because most endogenous components do not absorb or emit within the OCT illumination spectral range, and some exogenous agents are available outside that range. If spectral overlap occurs, LIF and OCT need to be time or spatially multiplexed. Spectral overlap has been used to advantage in one application [128], with simultaneous OCT/ICG imaging in the eye’s fundus. In this case, the same superluminescent diode (793 nm center wavelength, 22 nm spectral full width at half maximum) was used for OCT imaging and for excitation of ICG. The key in this implementation was proper selection of the dichroic filter separating backscattered source light (directed to the OCT detector) from fluorescence emission (directed to the LIF detector). This selection minimized distortion of the source correlation function and maximized collection efficiency of fluorescence emission. Excellent simultaneous OCT and ICG images were obtained. However, use of the same source for OCT and fluorescence excitation could become increasingly difficult as either the source bandwidth increases or fluorophore emission intensity decreases, unless very large Stoke’s shift NIR-excitable agents become available.
A strategy to provide greater spectral separation has been described [129]. This custom system included a mode-locked Ti:sapphire laser and a 0.9 numerical aperture water immersion objective to generate simultaneous en face optical coherence and two-photon-excited fluorescence images. An image of a green fluorescent protein expressing drosophila embryo was presented; presumably endogenous fluorophore distribution could be imaged as well. Because fluorescence occurred at a much shorter wavelength range, no difficulties were encountered separating the fluorescence from the backscattered light. However, implementation of this method in an endoscopic fashion would be technically challenging because of high peak-power needs, three-dimensional scanning requirements, and the need to control probe–tissue separation.
50.5.4 Materials
As described above, many interesting endogenous fluorophores are best excited at ultraviolet wavelengths that also cause autofluorescence in most optical materials. Many glasses and polymers exhibit autofluorescence that is similar in spectral shape and range to the fluorescence of endogenous tissue fluorophores [130]. Because probe autofluorescence can easily become significant compared to the tissue signal, each component of the OCT–LIF system should be considered for autofluorescence, particularly if the excitation wavelength lies in the UV. The location of the element within the system is equally important as the absorption and emission characteristics of the material. Autofluorescence from an optically thick piece of fiber is generally more significant than from the same material used in a thin window. Additionally, isotropically emitted fluorescence is more easily collected from an element located near focus than from a lens or window located far from focus or in a low-numerical-aperture portion of the beam path. Lenses and windows made of fused silica and CaF2 have low fluorescence under UV-A excitation and good NIR transmission and are recommended throughout the beam path. Thick windows near the tissue should be restricted to lowest fluorescence glasses, although very thin windows of other glasses and even some low-fluorescence plastic films may be acceptable [131]. Usually, some fluorescent materials must be used despite the best attempts to avoid them. For example, the emission from even “low-fluorescence” ultraviolet transmitting optical epoxies is significant, and care should be taken to keep glue joints thin [132]. The jacketing materials available on many commercially available communications grade fibers ideal for OCT fluoresce strongly. Although fiber choice could be restricted only to low-fluorescence jacketed materials, it is often more practical to simply shield the fiber from UV-A excitation. This approach of hiding the fluorescent material behind absorbent material, a dichroic filter, or simply out of the beam path is the most flexible and commonly used. Care should be taken with items outside the device that may also autofluoresce, including tissue index matching gels, preservation media, culture plates, or wax mounting blocks.
50.5.5 Safety
The tip of a combined OCT–LIF endoscope is either in contact with or at a close distance to a tissue surface or body fluid and therefore should be analyzed for potential hazards. A thorough analysis of potential risks and protection against those risks are a requirement for human subject studies. Hazards include electrical shock hazards, clinical hazards, material toxicity hazards, and radiation hazards. Of unique importance to combined OCT–LIF systems is the fact that both systems may be operated concurrently, requiring cumulative radiation exposure analyses. Radiation exposure in the UV poses a different hazard than in the visible/NIR. UV exposure is a cumulative hazard due to the potentially ionizing energy of each photon (exposure limits can be calculated for repeated exposure over days), whereas visible and NIR light exposure is analyzed for potential thermal injuries. “Blue-light hazard” is unique to eye exposure, caused by free radical release due to interaction with visual photopigments [133]. Threshold limit values for radiation exposure are defined by several standardization organizations: The American National Standards Institute (ANSI) publishes maximal permissible exposure levels [134]; the American Conference of Governmental Industrial Hygienists (ACGIH) publishes similar threshold limit values (TLV) and biological exposure indices [135]. Applications which intentionally introduce light into the eye should verify that standards set for ophthalmic instruments ISO 15004-2 are met in addition to laser safety standards. In contrast to the above-listed standardization organizations, the International Commission on Non-Ionizing Radiation Protection (Oberschleissheim, Germany) makes their guidelines available online at no cost and publishes them in Health Physics. Usually the threshold limit values are expressed for laser beam [136, 137] and incoherent exposure [138, 139] of the eye and skin. For broadband UV exposure (<400 nm), the biologically effective radiation (device emission weighted by the biologic action spectrum, which is normalized at 270 nm) should not exceed the TLV for melano-compromised skin and eye (3 mJ/cm2) [138]. The TLV for the skin and eye has also been recommended for the cervix in a guidance document developed by the US Food and Drug Administration’s Center for Device and Radiological Health (CDRH) [140]. For the wavelength range of 0.38–1.4 μm, exposure limits are governed by thermal injury to the skin and eye. Unfortunately, the authors are not aware of any studies evaluating combinational effects of NIR radiation and UV exposure.
50.5.6 Optical Design Considerations
The optical design of a combined system is a result of an attempt to optimally control the photon paths in the tissue for each modality, while meeting material constraints in the optical path and separating the signals (chromatically, spatially, or temporally) to the appropriate detector. The useful signal in OCT depends on photons that undergo a single scattering event in a nearly backscattering direction. The confocal design of typical OCT systems helps reject photons that take any other path. Lateral resolution is dependent upon the beam cross section at a given depth. Usually, a balance between lateral resolution and depth of focus is struck in order to avoid axially scanning the objective [141]. Conversely, LIF photons are typically allowed to take a circuitous path: the excitation light may be scattered multiple times before it is absorbed. If fluorescence conversion occurs, the emission spectrum of the intrinsic fluorescence will be modified as the emitted photons wander randomly through the tissue until they are absorbed by chromophores or released at the tissue surface. The path taken, and volume subsequently probed by the LIF system, depends on the tissue absorption and scattering properties as well as factors determined by the probe geometry: the insertion angles of excitation photons, the distance along the surface the collection to point (source-detector separation distance – SDSD), and the angles over which the photons are collected. Fiber-optic probes have been proposed for preferential depth-sensitive fluorescence sensing with the use of variable illumination and collection apertures [142, 143], illumination collection distances [143–146], and illumination collection angles [147–149].
Monte Carlo analysis of specific fiber-optic probe implementations on media with tissue-like optical properties and their associated experimental verifications show trends that are useful in designing an LIF probe. First, it is critical to control the distance between the illumination/collection fibers and the tissue for quantitative tissue analysis [145, 150]. When the illumination and collection areas are superimposed at the tissue surface (SDSD = 0), either by means of single illumination–collection fiber in contact with the tissue, or a spacer distal to a group of fibers that allows the illumination–collection areas to diverge over the same tissue area, the probe will be primarily sensitive to superficial layers less than 400 μm deep [142, 144, 145]. Similarly shallow tissue sampling can be achieved when source and collection fibers are separated at the tissue surface but are inclined towards each other to cause an overlap of the fiber numerical apertures in the superficial tissue [148, 149, 151]. The average probed depth can be increased from 700 to 1,200 μm by placing normally incident illumination and collection fibers that are directly in contact with the tissue at increased separation [142, 144, 145]. Evidence with diffuse reflectance modeling suggests that the probed depth can be increased yet further by inclining the fibers away from each other [152]. In general, greater overlap of the numerical apertures of the illumination and collection fibers improves collection efficiency while the expected probing depth decreases. The lateral extent of a collected LIF photon’s path has been relatively unexplored, although lateral resolution somewhat larger than the SDSD could be expected. Increasing the fiber core diameter and numerical aperture increases the light gathering capacity of the collection fiber but may have effects on the depth of collected fluorescence that depends upon the configuration. In cases with fibers in contact with the tissue, the size of the illumination and collection area introduces a superposition of many SDSDs, while the numerical aperture of the fiber introduces a superposition of many angles of insertion and collection. In addition to the depth selectivity provided by SDSD, some geometries appear to be optimally sensitive to fluorophore distribution rather than scattering properties of the tissue [153, 154]. Knowledge of tissue layer thicknesses from OCT images could help in the selection of optimal LIF illumination/detection fiber configurations.
In the case where the majority of information from LIF derives from direct emission from a fluorophore in a relatively superficial tissue layer, a confocal arrangement delivers significant advantages. Confocal arrangements are common in fluorescent microscopes because they have the potential to deliver high-resolution imaging with high contrast. High resolution derives from the tightly limited photon paths allowed by the confocal arrangement. Contrast is enhanced by spatial filtering of out-of-plane, unwanted fluorescence, including that from the optics of the instrument itself. In combination with point-scanning OCT, the two confocal modalities will share similar design needs and constraints.
Because OCT systems generally utilize single-mode fibers and LIF systems utilize multimode fiber (to optimize the collected fluorescence emission signal), the most straightforward OCT–LIF endoscope design utilizes separate fibers for each modality. Maintaining separate conduits for OCT and LIF minimizes the cross talk between the systems and helps maintain high signal-to-background ratio. The proximal coupling optics between the fibers and sources/detectors are also simplified. However, other fiber configurations are possible. Dual-clad fibers consist of a central core with two layers of cladding. The core can be made single mode, whereas the inner cladding has a large radius and high-numerical aperture (multimode). OCT light is channeled to the sample through the core. LIF excitation light can be carried by the core (if compatible) or the inner cladding. Remitted OCT, excitation, and emission light are coupled into the core and inner cladding. In addition to coupling light into the dual-clad fiber, the proximal optics must spatially separate returned OCT light which has propagated through the core from that which has taken unwanted paths through the inner cladding, and spectrally separate the OCT and fluorescence emission. Dual-clad fibers have successfully been used in OCT–LIF systems [155–158], and one system is described in more detail below.
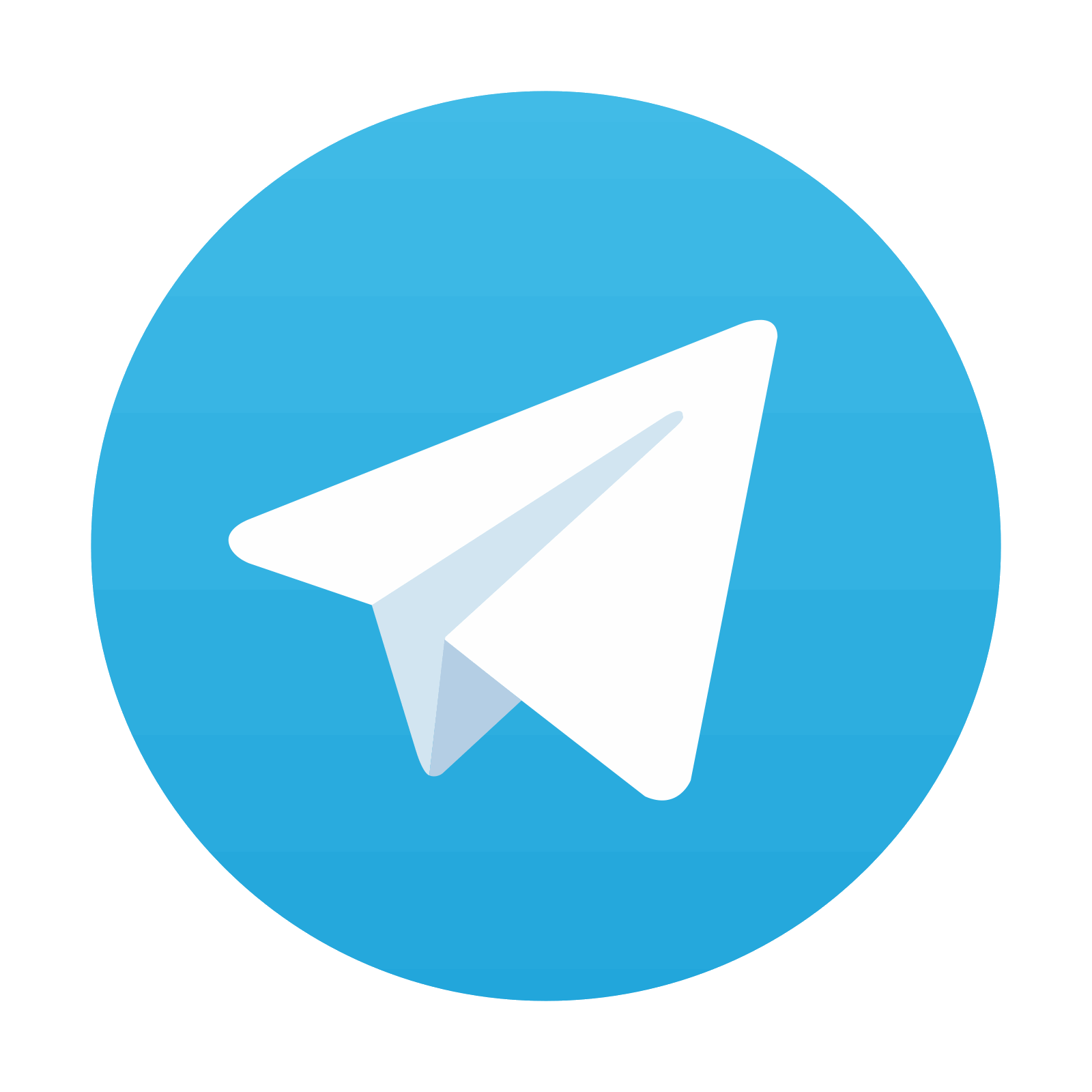
Stay updated, free articles. Join our Telegram channel
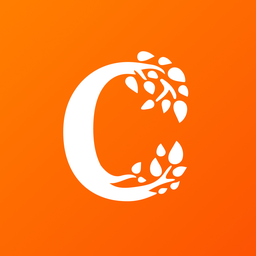
Full access? Get Clinical Tree
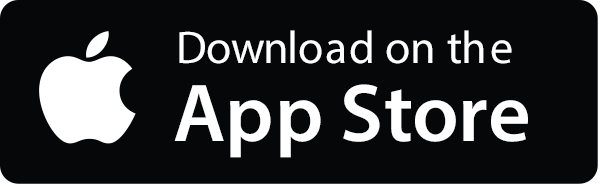
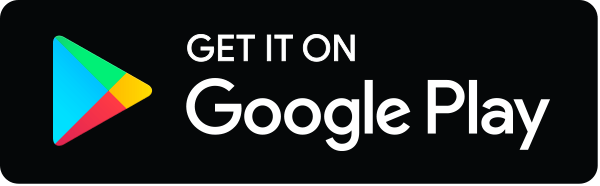