Fig. 46.1
In OCT, a probe beam is reflected from a sample. In a moving sample such as cells in a blood vessel, the frequency of reflected light is Doppler shifted in proportion to the component of the flow velocity in the direction of beam propagation
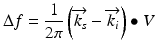
(46.1)
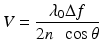
(46.2)
In FD-OCT, this frequency shift Δf will introduce a phase shift in the spectral interference pattern that is captured by the line camera. With fast Fourier transform (FFT), the transformation result is a complex function characterized by amplitude and phase. The phase difference between sequential axial scans at each pixel is calculated to determine the Doppler shift [3]. One limitation of phase-resolved flow measurement is an aliasing phenomenon caused by 2π ambiguity in the arctangent function. This phenomenon limits the maximum determinable Doppler shift to Δf = 1/(2τ), where τ is the time difference between sequential axial lines. Thus, the maximum detectable speed is V = λ 0/(4nτ cosθ).
Using Eq. 46.2 to determine real flow speed V, the Doppler angle θ must be determined. Therefore, we needed at least two locations on a same vessel to decide the vessel vector.
46.2.2 Circumpapillary Double-Circular Scan
In the circumpapillary double-circular scan pattern, the OCT sampling beam scans two circles around the optic disc (Fig. 46.2), which ensures we sample at least two points for any main vessel around the optic disc. With a 26 kHz FD-OCT, the frame rate was six frames per second. Each scan contained 12 circles and was recorded over consecutive 2-s intervals (Fig. 46.2a).
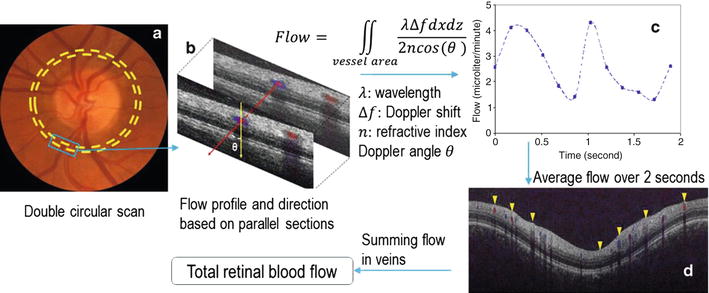
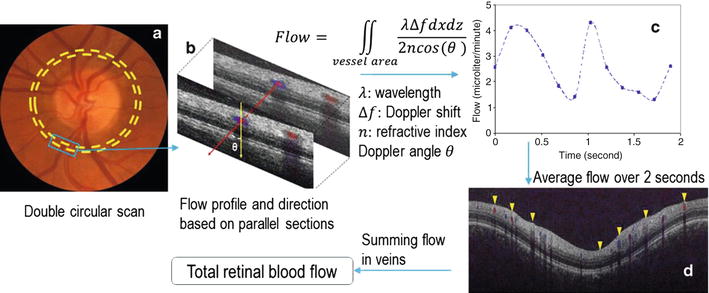
Fig. 46.2
Measurement of retinal blood flow using double-circular Doppler scan. (a) Two concentric circular scans transect all retinal blood vessels emanating from the optic nerve head (ONH). (b) The blood vessels are identified by Doppler frequency shift within the lumen as shown by blue and red color Doppler displays. By comparing the lumen positions in the two concentric sections, the vessel orientation relative to the OCT beam is measured. Velocity is calculated using the Doppler shift and Doppler angle. Volumetric blood flow rate is calculated by integrating velocity within the lumen area. (c) Flow measurements are averaged from 12 circular scans recorded over 2 s. (d) Total retinal blood flow (TRBF) is calculated by summing flow from all detected veins
Figure 46.2b shows the three dimensional diagram of the scan pattern in the rectangular region of Fig. 46.2a. The two positions of the blood vessel segment on the two scanning circles have coordinates P 1(r 1 , α 1 , z 1 ) and P 2(r 2 , α 2 , z 2 ), respectively. The vector of the blood vessel can be expressed as r b (Δx = r 1 cosα 1 − r 2 cos α 2, Δy = r 1 sinα 1 − r 2 sinα 2, Δz = z 1 − z 2). For OCT scans at radius r, when the probe beam scans to the angle α, the vector of the scanning beam is s (r cosα, r sinα, –h), where h is the distance from the nodal point to the retina. With vector s and r b , the Doppler angle θ between the OCT probe beam and vessel can be calculated.
46.2.3 Correction for Sampling Density Effect
In phase-resolved Doppler OCT, the phase difference between sequential axial scans is calculated to determine Doppler frequency shift. If the sampling locations are not negligibly small relative to the beam diameter, phase decorrelation results in a decrease in the measured Doppler shift. For example, in the dual circular scan pattern, there are 4,000 axial lines for each circle. At a scanning radius of 1.8 mm, the sampling step is about 2.8 μm. For the FD-OCT with laser beam of 20 μm focus spot size, a calibrated factor of 0.8 is needed to correct the measured flow result.
46.2.4 Correction of Bulk Motion
The Doppler information contains artifacts from the motion of the eye and the OCT system. To obtain the Doppler frequency shift induced by actual blood flow, the artifacts must be identified and subtracted. The eye and OCT motion artifacts induce constant Doppler shift for whole axial scan (A-scan). Therefore, most bulk motion correction methods calculate the constant phase term of each a-scan and subtract it from the Doppler shift [19–21].
Our study showed that bulk motion is equivalent to the average Doppler signal between the inner limiting membrane and the top vessel boundary for each a-scan. This value represents the Doppler signal due to local tissue motion and is subtracted from the Doppler signal in the whole a-scan to get the net signal induced by blood flow.
46.2.5 Correction of Phase Wrapping
For FD-OCT systems with 840 nm laser source and 26 kHz scan rate, the maximum measurable axial velocity component in the eye is 4.2 mm/s. On the other hand, a typical average velocity in the main vein is about 18 mm/s and the velocity in the center of the vein is about 36 mm/s. In order to detect Doppler shifts without phase wrapping, the Doppler angle needs be above 82°. In order to allow measurement of vessels with smaller Doppler angles, a phase-unwrapping algorithm is needed. Figure 46.3 shows the Doppler signal with onefold phase wrapping (maximum Doppler shift was between π and 2π) and after phase unwrapping for a vessel.
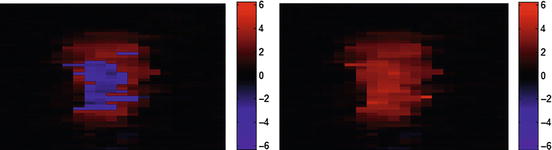
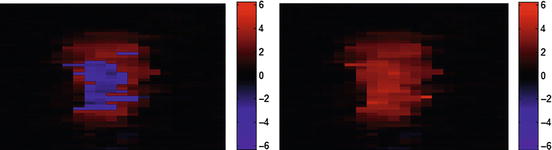
Fig. 46.3
Phase unwrapping in a single vessel. Left: Doppler shift with phase wrapping. Right: Doppler shift after phase unwrapping
However, only onefold of phase unwrapping is practical. Multiple phase unwrapping is difficult in practice because of noise. Therefore, it is difficult to get accurate measurements of arteries with high velocities. In our study, the total TRBF is measured from only from veins.
46.2.6 Reduce Eye Motion Error for Doppler Angle Estimation
The measured position of vessels contains noise from eye motion. Variance is doubled when we use two positions for vessel vector estimation, and this dominates the variance of the Doppler angle measurement and flow calculation. To reduce the eye motion artifact for vessel position, the circles are registered in transverse direction based on vessel pattern matching. Vessel vectors between consecutive circles are then averaged to reduce the eye motion error.
46.2.7 Correction for Pulsation
In a cardiac cycle, the flow velocity changes with pulsation. In order to get an average flow velocity, it is necessary to average flow at different time points. In CDCS, the Doppler shift in a vessel is averaged from the 12 frames, which corresponds to 12 time points in 2 s.
46.2.8 Semiautomated Doppler OCT Grading Software
We developed a software, which we named “Doppler OCT of retinal circulation,” or DOCTORC, to include all the above ideas for measuring TRBF [22]. DOCTORC can automatically detect vessels on all circles and calculate the Doppler angle and TRBF. It can also provide total vessel area and average vessel velocity measurements. In the event of incorrect vessel detection, DOCTORC allows for human input to manually correct the error.
DOCTORC identifies blood vessels based on both Doppler and reflectance OCT images. The distinction between veins and arteries is based on both OCT images and fundus photographs. Vessel diameter (D) is measured by computing caliper on the cross-sectional Doppler OCT images which is used to compute lumen area (πD 2/4). The venous cross-sectional areas for all branch vessels around the optic disc are summed to obtain the total venous area for the eye. The Doppler angle between the vessel and OCT beam is measured by the relative position of each vessel in two concentric OCT images.
The effect of eye motion on the calculation of Doppler angle is small due to the short time interval between the inner and outer circular scans (0.16 s). This error is further minimized by averaging the Doppler angle estimates from concentric circular scans. Flow velocity can be computed from the Doppler shift and Doppler angle, with steps to account for the effect of background retinal motion and transverse scan step size [23]. When the peak axial Doppler shift is between π and 2π (or −π and −2π) at the center of the vessel, the phase-unwrapping algorithm previously described is automatically applied to allow valid flow measurements [24].
Veins are identified by the flow direction toward the optic disc. The volumetric blood flow rate for each pixel is calculated by multiplying the velocity by vessel area. Flow within a vein is calculated by summing the flow in the pixels over lumen cross section. Flow measurements are averaged over each 2-s recording. Measurements from all valid scans are averaged. Total retinal blood flow is calculated by summing flow from all detectable veins. Retinal blood flow in arteries and veins should have an equal sum because inflow must equal outflow in any steady state system that obeys the law of conservation of mass. This has been confirmed by actual measurements of retinal arterial and venous flows with a number of techniques [25]. Thus, measuring total venous flow alone is sufficient to quantify the total retinal blood flow. Average venous velocities are obtained by dividing the total retinal flow by the total venous areas.
46.2.9 Blood Flow Measurement for Normal and Glaucoma with Doppler OCT
We performed a study comparing blood flow measurements obtained with Doppler OCT between glaucoma patients and normal subjects. A total of 47 consecutive eyes of 42 patients with glaucoma were age-matched with 27 normal eyes of 27 patients [23]. There were no significant differences in age, systemic hypertension, and diabetes mellitus between the two groups. However, the mean intraocular pressure was lower in glaucoma eyes (p = 0.03), which were all undergoing treatment.
Traditional measures of function and structure were consistent with expected findings for glaucoma eyes (Table 46.1). Glaucoma eyes demonstrated visual field loss with lower mean deviation (MD) values and higher pattern standard deviation (PSD) values compared to normal eyes (p < 0.0001). Structural evaluation identified loss of disc rim area, GCC thickness, and RNFL thickness in glaucoma eyes relative to normal eyes (p < 0.0001).
Table 46.1
Visual field and Doppler OCT measurements in normal and glaucoma subjects
Parameter | Normal | Glaucoma | p-value |
---|---|---|---|
Visual field | |||
Mean deviation (dB) | 0.16 ± 1.00 | −4.39 ± 4.14 | <0.0001 |
Pattern standard deviation (dB) | 1.61 ± 0.39 | 6.54 ± 4.45 | <0.0001 |
Total retinal blood flow (μl/min) | 45.5 ± 9.5 | 34.9 ± 8.5 | <0.001 |
Arterial area (mm2) | 0.033 ± 0.0077 | 0.028 ± 0.0074 | 0.006 |
Venous area (mm2) | 0.047 ± 0.012 | 0.041 ± 0.0086 | 0.01 |
Arterial velocity (mm/sec) | 23.9 ± 7.2 | 21.8 ± 7.3 | 0.22 |
Venous velocity (mm/sec) | 16.3 ± 2.8 | 14.5 ± 3.7 | 0.03 |
Total retinal blood flow was significantly reduced in glaucoma eyes with decreased vessel area and venous velocity compared to normal eyes (Table 46.1). Blood flow reduction was correlated with visual field loss, but had no correlation or paradoxical correlation with structural loss of neural tissue as measured by optic disc rim area, peripapillary nerve fiber layer (NFL) thickness, or macular ganglion cell complex (GCC) thickness (p ≥ 0.23). Univariate analysis demonstrated that each dB decrease in blood flow resulted in a 1.91 dB decreased in MD (p < 0.001). Multivariate analysis confirmed a largely independent relationship between reduced blood flow and visual field loss, even after accounting for structural loss of rim area and NFL thickness.
46.2.10 Blood Flow Measurement in Diabetic Retinopathy and Optic Neuropathy Eyes with Doppler OCT
The Doppler OCT and algorithms were also applied to nine eyes with nonarteritic ischemic optic neuropathy (AION), five eyes with proliferative diabetic retinopathy (PDR, after treatment with pan-retinal photocoagulation), and two eyes with branch retinal vein occlusion (BRVO) [26]. All diseases caused significant reduction of total blood flow compared to normal (AION, 28.2 ± 8.2 μl/min; PDR, 15.8 ± 10.1 μl/min; BRVO, 26.7 ± 5.7 μl/min).
46.3 OCT Angiography
Several OCT-based techniques have been successfully developed to image microvascular networks in human eyes in vivo [9, 24, 25, 27–32]. One example is optical microangiography (OMAG), which works by using a modified Hilbert transform to separate the scattering signals from static and moving scatters [33]. By applying the OMAG algorithm along the slow scanning axis, high-sensitivity imaging of capillary flow can be achieved [34]. However, the high sensitivity of OMAG requires precise removal of bulk motion by resolving the Doppler phase shift [35]. Thus, it is susceptible to artifacts from system or biological phase instability. Other related methods such as phase variance [25] and Doppler variance [31] have been developed to detect small phase variations from microvascular flow. These methods do not require non-perpendicular beam incidence and can detect both transverse and axial flow. They have also been successful in visualizing retinal and choroidal microvascular networks. However, these phase-based methods require precise removal of background Doppler phase shifts due to the axial movement of bulk tissue. Artifacts can also be introduced by phase noise in the OCT system and transverse tissue motion, and these also need to be removed. In order to circumvent these issues, our research group has investigated the use of amplitude-based OCT signal analysis, which in this context may be advantageous for ophthalmic microvascular imaging.
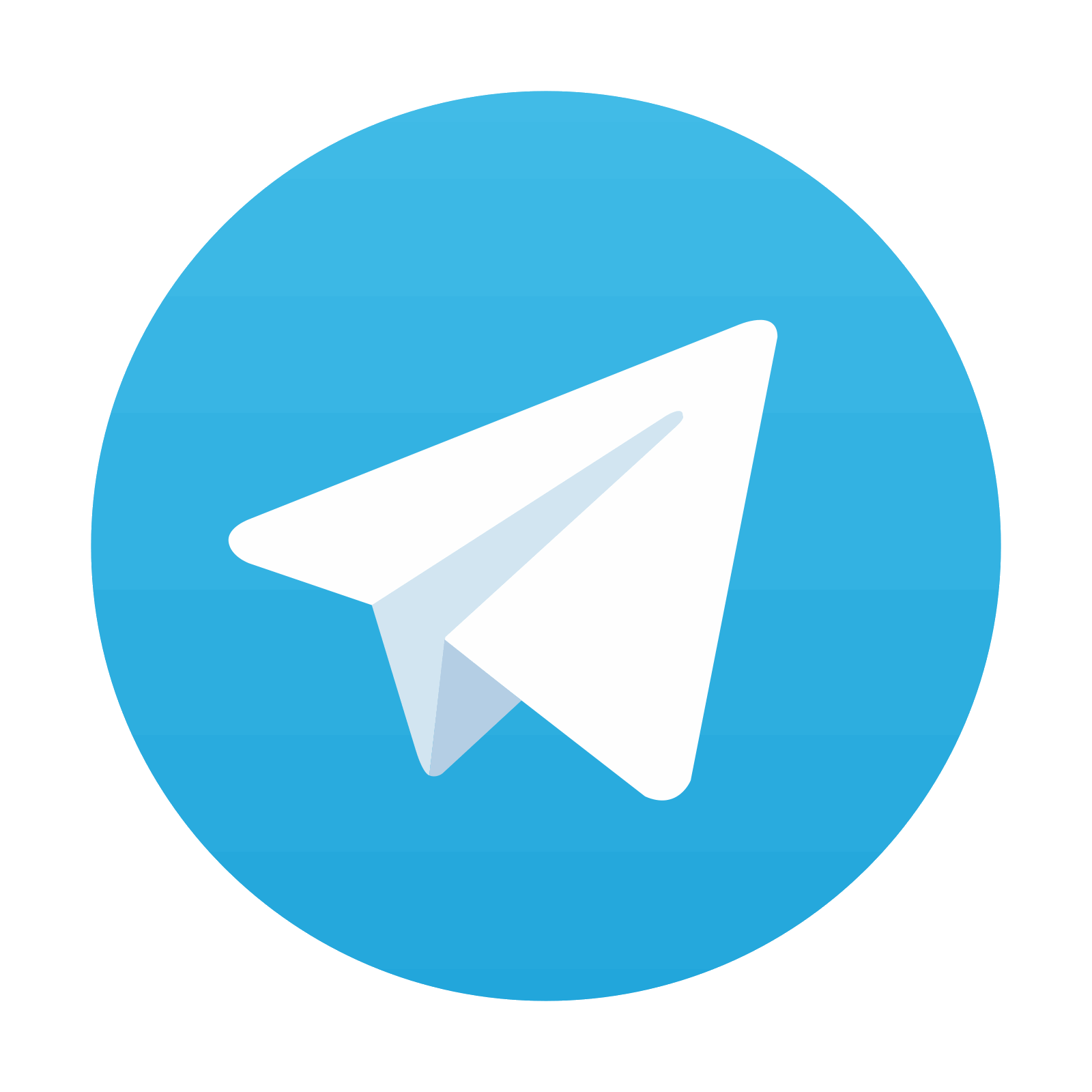
Stay updated, free articles. Join our Telegram channel
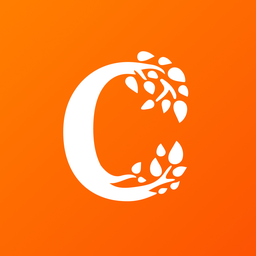
Full access? Get Clinical Tree
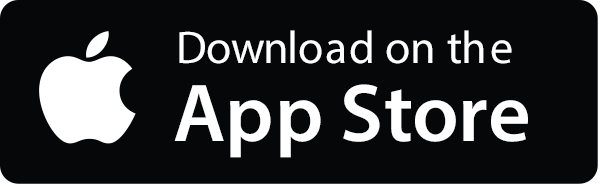
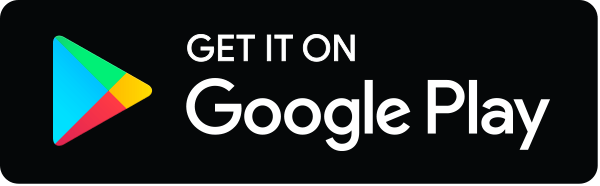