Fig. 6.1
Schematic drawing of spiral ganglion neurons and their central and peripheral terminations. The type I neuron (black) innervates a single IHC and projects in a topographic fashion into the CN. Fibers that innervate the basal hair cells project to dorsal regions of the CN, whereas fibers that innervate more apical hair cells project to progressively more ventral regions. This type I neuron is representative of its group. Note that a representative type II neuron (red) has a similar central projection pattern but with additional terminations in the GCD. The conundrum is that in spite of the differences in peripheral innervation, the central projections have a similar spatial pattern (Adapted from Brown et al., 1988)
Morphological generalizations such as cell body size or shape (Berglund & Ryugo, 1987) may not always be sufficient in every species for distinguishing between spiral ganglion cell types. In such instances, the ratio of the diameter of the central and peripheral processes of spiral ganglion neurons, when measured in proximity to the soma, is informative (Kiang et al., 1982; Berglund & Ryugo, 1987). The calibers of both processes of type II cells are generally comparable, whereas the peripheral diameter of type I cell processes is characteristically much smaller than the central counterpart. This observation has been reported in a number of species including the cat, mouse, opossum (Didelphimorphia), guinea pig (Cavia porcellus), squirrel monkey (Saimiri sciureus), and human (Kiang et al., 1982, 1984; Berglund & Ryugo, 1987).
A number of staining techniques can also be employed to distinguish between spiral ganglion cell populations. Because of different cytoskeletal profiles, type II neurons can be selectively labeled using antibodies directed against the phosphorylated 200-kDa neurofilament protein (Berglund & Ryugo, 1986, 1991), or peripherin, a neuronal intermediate filament protein (Hafidi et al., 1993). It is also possible to label type I neurons rapidly and selectively using dextran-based neuronal tracers by applying dye crystals directly onto the freshly sectioned auditory nerve (Huang et al., 2007).
6.2.2 Physiology
Auditory nerve fibers exhibit a diverse range of intrinsic and evoked physiological response profiles (reviewed by Davis and Crozier, Chap. 4). Such variety in the afferent input to the central auditory system highlights the complexity of coding strategies used by the brain to analyze the sound environment. The physiological properties of type I spiral ganglion neurons have been well characterized by recordings from their axons (Kiang et al., 1965; Evans, 1972). In contrast, virtually nothing is known about the in vivo response properties of type II cells because of their scarcity and fine axon caliber (Liberman, 1982a; Robertson, 1984). Recent progress has been made with recordings at type II afferent terminals under OHCs (Weisz et al., 2009, 2012), but how this information is functionally integrated at the type II soma and propagated to the central nervous system remains undetermined.
Type I fibers can be differentiated on the basis of three basic physiological properties: spontaneous discharge rate, response threshold, and characteristic frequency. Spontaneous discharge rate (SR) describes the firing activity of an individual nerve fiber in the absence of sound stimulation. The response threshold of auditory nerve fibers refers to the lowest sound pressure level that elicits a supra-threshold response (e.g., a firing rate 10 % above SR or an increase of one spike/stimulus above SR), typically measured in decibels (dB) relative to 20 µPa. Variations in threshold criteria, however, do not substantially change population groupings (Liberman, 1978; Geisler et al., 1985). Lastly, the characteristic frequency (CF) is defined as the sound frequency at which an individual auditory nerve fiber is most sensitive. The CF is determined by measuring a “tuning curve” (Kiang et al., 1965; Evans, 1972), which describes the collection of frequency and intensity combinations that evoke increases in the firing rate above SR of an auditory neuron. The tip of this excitatory tuning curve indicates both the response threshold and CF of the unit.
6.2.2.1 Characteristic Frequency
The mammalian cochlea acts as a biological frequency analyzer of sound, with a low-to-high frequency gradient established from the apex to the base of the cochlear spiral (von Békésy, 1960). Because each radial fiber contacts only a single IHC, the CF of each type I cell is determined by the position of its peripheral innervation along the basilar membrane (see also Rutherford and Moser, Chap. 5). This systematic relationship was demonstrated by physiologically characterizing and tracing labeled auditory nerve fibers back to their cochlear origin, establishing a place-frequency map of the cochlea. Such experiments have been performed in a variety of mammals including cat, rat, opossum, gerbil, guinea pig, chinchilla, mouse, and bat (Liberman, 1982b; Kössl & Vater, 1985; Vater et al., 1985; Müller, 1991, 1996; Müller et al., 1993, 2005, 2010; Tsuji & Liberman, 1997; Muniak et al., 2013).
The determination of cochlear place-frequency maps also shows that, in most species, frequency representation is approximately log-linear, giving equal weighting to frequency components across the physiological hearing range. Deviations from this log-linear trend are seen in some species, where frequency expansions—termed acoustic foveae—for high frequencies used in echolocation have been observed in horseshoe (Rhinolophus rouxi) and mustache (Pteronotus parnellii) bats (Bruns & Schmieszek, 1980; Kössl & Vater, 1985; Vater et al., 1985), and in the low-frequency region of the African mole rat (Cryptomys hottentotus) cochlea (Müller et al., 1992). Whether specialized or generalized, the place-frequency map of the cochlea and its transfer to auditory nerve fibers establishes the range and sensitivity of hearing capabilities of the animal (Fay, 1988).
Type I spiral ganglion somata are distributed throughout Rosenthal’s canal with respect to CF (Keithley & Schreiber, 1987). Cells with low CFs are located apically, whereas cells with progressively higher CFs can be found at progressively more basal regions. Hence, order is maintained in the connection between peripheral targets and cell bodies. An inverse relationship of unknown significance has also been observed between type I soma size and CF in the cat. Low-CF neurons exhibit the largest somatic silhouette area but soma size becomes progressively smaller with increasing CF until plateauing at approximately 4 kHz (Liberman & Oliver, 1984).
6.2.2.2 Spontaneous Discharge Rate and Threshold
Type I spiral ganglion neurons exhibit a broad range of SRs, which have been strongly correlated with response threshold (Kiang et al., 1965; Liberman, 1978). Cells with high SRs consistently display low thresholds for activation, whereas cells with low SRs tend to have higher thresholds. Across the audible frequency range, units with similar CFs can vary in SR from near 0 to greater than 100 spikes per second. A bimodal distribution of SRs is frequently observed in cats (Kiang et al., 1965; Liberman, 1978; Evans & Palmer, 1980) and guinea pigs (Tsuji & Liberman, 1997). In these species, 60–70 % of fibers have high SRs (>30 spikes/s), and the remaining 30–40 % have low SRs (<10 spikes/s). The low-SR population can be further subdivided into low-SR (<0.5 spikes/s) and medium-SR (>0.5 spikes/s) units. In gerbils, rats, and mice, a clear bimodal distribution of SRs is not observed (Schmiedt, 1989; Ohlemiller & Echteler, 1990; el Barbary, 1991; Taberner & Liberman, 2005). The inverse relationship between SR and response threshold, however, remains constant across CF, suggesting a general organizational feature of the auditory nerve.
The distinction of type I fibers on the basis of SR is also reflected in their peripheral anatomy. High-SR fibers are larger in caliber, and almost always contact the pillar side of IHCs (Liberman, 1982a; Liberman & Oliver, 1984; Merchan-Perez & Liberman, 1996). In contrast, low- and medium-SR fibers are smaller, and contact only the modiolar side of the IHC, indicating that differences in SR may partially stem from differences in their afferent innervation (Rutherford and Moser, Chap. 5). This segregation of fibers is maintained within the osseous spiral lamina, with high-SR fibers traveling closer to the scala tympani, and low- and medium-SR fibers residing closer to the scala vestibuli (Kawase & Liberman, 1992; Tsuji & Liberman, 1997). A similar pattern of divergence for type I somata within the spiral ganglion is also observed with respect to SR, although high-SR neurons tend to be found throughout the canal (Kawase & Liberman, 1992). Such a separation was not found in the guinea pig (Tsuji & Liberman, 1997). The dissociation of type I auditory nerve fibers on the combined basis of SR, response threshold, and peripheral innervation strongly suggests that these disparate fiber classes may play separate roles in auditory perception.
6.3 Central Projections
The challenges of auditory coding confronted 20th-century physiologists such as Rafael Lorente de Nó (1933a, b, 1976, 1981), who marveled at the complicated nature of the task. He proposed that clues to understanding the physiology of hearing would be aided by anatomical research that described the distribution pattern of auditory nerve terminals and the cell types that received its inputs. He boldly declared that individual auditory nerve fibers were modular, with each fiber resembling the other and differing only in its origin in the cochlea. Moreover, he concluded that each fiber innervated every one of the 13 regions he described for the CN, contacting hundreds of the 40–50 neuron types (Lorente de Nó, 1933b). When Lorente de Nó (1937) described three patterns of ganglion cell innervation of the sensory receptors, he complicated the idea that divergent patterns of sensory receptor innervation would produce identical connections in the brain. As new data emerged, some of the major conclusions of Lorente de Nó have been abandoned but his observations have contributed significantly to our understanding of the auditory system and set the stage for modern hypothesis testing.
6.3.1 Auditory Nerve
The centrally projecting axons of spiral ganglion cells collect along the central axis of the cochlea within the modiolus. The total number of fibers contained within this bundle varies with species, ranging from 10,000 to 12,000 fibers in mice, 30,000 in humans, and 95,000 in dolphins (Guild et al., 1931; Rasmussen, 1940; Gacek & Rasmussen, 1961; Wever et al., 1971; Anniko & Arnesen, 1988; Nadol, 1988; Berglund & Ryugo, 1991; Chen et al., 2010; see Table 1 of Nayagam et al., 2011). Irrespective of absolute counts, the proportions of 90–95 % thick, myelinated type I fibers and 5–10 % unmyelinated type II fibers remain fairly constant (Alving & Cowan, 1971; Arnesen & Osen, 1978; Anniko & Arnesen, 1988).
Early electrophysiological experiments suggested that fibers with similar CFs were located near each other within the trunk of the auditory nerve (Kiang et al., 1965). Anatomical work confirmed this observation, showing an orderly arrangement of fibers within the modiolus from the spiral lamina through to the CN (Fig. 6.2; Sando, 1965; Arnesen & Osen, 1978; Anniko & Arnesen, 1988). Low-CF fibers originating from the apex of the cochlea are positioned within the central axis of the modiolar trunk. Fibers from more basal regions join the nerve bundle peripherally, progressively wrapping around its outer edge. This spatial pattern within the nerve forms a helical twist, echoing that of the cochlea. The arrangement spirals through the internal auditory meatus and continues into the central nervous system by crossing the Schwann–glia border, where the bundle begins to unwrap as auditory nerve fibers make their topographic projections into the CN (Sando, 1965; Arnesen et al., 1978; Muniak et al., 2013).
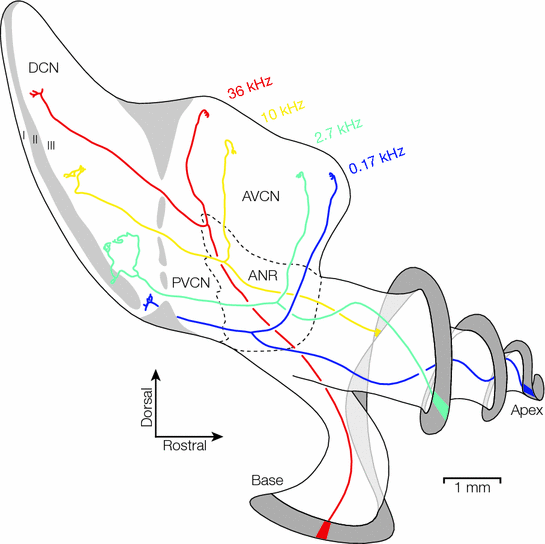
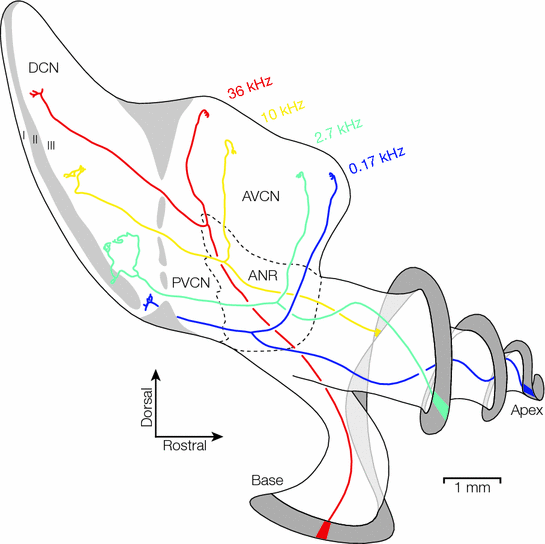
Fig. 6.2
Schematic diagram of the CN complex and cochlear nerve in cat, as shown from a dorsolateral aspect. The cochleotopic/tonotopic projections of four type I auditory nerve fibers are illustrated. Each fiber begins at the spiral lamina and twists through the cochlear nerve bundle to emerge in the auditory nerve root, where it bifurcates and gives rise to an ascending branch, which terminates within the AVCN, and a descending branch, which passes through the PVCN and terminates within layer III of the DCN. The position of each fiber along its entire path is frequency dependent. The auditory nerve root region is shown as a dotted outline, with the ventral boundary corresponding to the Schwann–glia border. The GCD and layer II of the DCN are indicated in gray. ANR, auditory nerve root; AVCN, anteroventral cochlear nucleus; DCN, dorsal cochlear nucleus; PVCN, posteroventral cochlear nucleus (Adapted from Arnesen & Osen, 1978; Ryugo & May, 1993)
6.3.2 Cochlear Nucleus
The CN is located on the dorsolateral aspect of the brain stem at the pontine–medullary junction. It is divided into a dorsal cochlear nucleus (DCN) and a ventral cochlear nucleus (VCN). These two divisions are visible externally, with a slight depression separating them along the lateral surface. The DCN is a laminated cortical structure reminiscent of a cerebellar folium (Ramón y Cajal, 1909; Lorente de Nó, 1933b). The internal organization of the VCN is less obvious. The two divisions are separated by a thin expanse of granule cells, which is visible in Nissl-stained sections (Mugnaini et al., 1980). The CN is the sole target of auditory nerve input (Fekete et al., 1984; Liberman, 1991). Individual auditory nerve fibers bifurcate on entering, and the zone of bifurcations roughly separates the VCN into an anterior and a posterior division (AVCN, PVCN; Ramón y Cajal, 1909). Cytoarchitectonic features such as size, shape, and packing density of cell bodies, dendritic branching, and fiber patterns can also be used to subdivide the nucleus (Harrison & Irving, 1965; Osen, 1969; Brawer et al., 1974). Different cell populations within these subdivisions may be further characterized by features of the cytology of the cell body, characteristics of the nucleus, afferent innervation, immunologic staining, axonal projections, and physiological response properties, but the borders between different populations are often fuzzy (for review, see Cant, 1992). Differential patterns of ascending projections from cells of the CN help to establish divergent representations of the sound environment along parallel central auditory pathways. The systematic and generally reliable relationship between anatomical and physiological properties suggests a role in stimulus coding and signal processing.
6.3.2.1 Type I Fibers
Auditory nerve fibers project into the CN following a stereotyped plan (Figs. 6.1 and 6.2; Ramón y Cajal, 1909; Lorente de Nó, 1933a). On crossing the Schwann–glia border, fibers ascend dorsally into the nucleus forming the root branch of the nerve. After traversing for some distance, the root branch bifurcates, giving rise to an ascending branch and a descending branch (collectively called “parent” branches). The ascending branch projects into the anterior region of the AVCN, where one of its most pronounced terminals is a large, axosomatic ending known as the endbulb of Held (Held, 1893). The descending branch is directed posteriorly through the PVCN and usually (85 % of cases in cats) continues onward and upward into the DCN (Fekete et al., 1984). Nodes of Ranvier can be observed at regular intervals along the parent branches, and the mean lengths of ascending and descending branches are similar irrespective of CF or SR (Fekete et al., 1984).
Each parent branch maintains a relatively straight trajectory and can give rise to short collaterals and multiple endings as they traverse through the nucleus. In the cat, the ascending branch produces an average of nine primary collaterals, and the descending branch gives rise to an average of 11 primary collaterals (Fekete et al., 1984). These collaterals are thinner, divide several times, exhibit en passant and terminal swellings, and generally do not extend far from the parent branch. En passant swellings do not consistently display synapses, but terminal swellings always do (Fekete et al., 1984; Ryugo & Sento, 1991). Collaterals are scattered along the length of each parent branch, resulting in a patchy terminal field for any given auditory nerve fiber. In the cat, approximately 15 % of terminal swellings reside in the DCN, with the remainder scattered throughout the VCN (Ryugo & May, 1993). Fibers sharing similar CFs (see Sect. 6.3.2.2) occupy the same region, thus filling in the patches to form a solid terminal field along the length of the projection (Fekete et al., 1984; Ryugo & May, 1993). All terminals, however, remain within the magnocellular core of the VCN and the deep layer (III) of the DCN (Fekete et al., 1984; Liberman, 1991).
6.3.2.2 Organization with Respect to Frequency
Topographic maps are one of the fundamental principles of brain organization, defined by an orderly representation of sensory and motor systems (Kaas, 1997). In the auditory system, frequency maps are conserved throughout the ascending pathways, replicating the place-frequency map established at the cochlear sensory epithelium (Clopton et al., 1974). Tonotopy is thus imprinted upon the CN via the precise distribution of auditory nerve fibers relative to CF (Fekete et al., 1984; Ryugo & May, 1993). This organization can also be referred to as cochleotopy, which describes the organization of projections with respect to their position of origin on the cochlear spiral. Because of the orderly organization of the cochlear place-frequency map (Liberman, 1982b), the concepts of tonotopy and cochleotopy are often interchangeable. This correspondence is useful for interpreting anatomical studies from which physiological data may not be available.
An electrophysiological study first established that a complete representation of audible frequencies could be found within the CN (Rose et al., 1959). Electrode penetrations along a dorsal to ventral axis revealed frequency tunings that progressed from high to low CFs. The range of CFs encountered on a particular electrode track varied with location, but similar trends were observed in both dorsal and ventral divisions. Subsequent physiological experiments have expanded upon this view, establishing that frequency tuning is highly organized within the DCN, AVCN, and PVCN (Bourk et al., 1981; Spirou et al., 1993). Spatial reconstructions of electrode penetrations in these studies were sufficiently detailed to support the conclusion that frequency representations were conceptually organized into isofrequency laminae.
Exploration of the cochleotopic projection pattern of the auditory nerve into the CN began with the histologically stained material of early neuroanatomists (Ramón y Cajal, 1909; Poljak, 1927; Lorente de Nó, 1933a). Initial experimental studies verified a rough correspondence between a lesion point in the cochlea and the position of the silver grains attracted by degenerating axons to identify the bifurcation of the root branch and the distribution of auditory nerve fiber projections (Sando, 1965; Osen, 1970; Webster, 1971). Apical parts of the cochlea, representing low CFs, projected to ventral regions of the CN, whereas basal, high-CF portions projected to dorsal areas. More direct results were obtained following small extracellular tracer deposits made in the cochlea that filled the axons and their terminations in the CN (Leake & Snyder, 1989; Brown & Ledwith, 1990). Another study involved small injections into defined frequency locations of the CN that backfilled auditory nerve fibers within the nucleus as well as peripheral terminations in the cochlea (Muniak et al., 2013). When analyzed in the coronal plane, these injections produced “slabs” of labeled fibers that coursed throughout the rostro-caudal extent of the nucleus. The dorsoventral position of these slabs was cochleotopic, giving an anatomical substrate to the isofrequency laminae observed electrophysiologically.
Further refinement of these findings was obtained through the reconstruction of physiologically characterized and intracellularly labeled auditory nerve fibers in the cat (Fekete et al., 1984; Liberman, 1991; Ryugo & May, 1993). Single-fiber studies not only provide excellent resolution for analyzing the fine details of primary projections but also yield unambiguous structure–function relationships. These results provided unequivocal evidence that the position of bifurcation of the root branch and the spatial distribution of the central axon is strongly correlated with the CF of the fiber (Fig. 6.2). Fibers with low CFs bifurcate almost immediately on entering the CN, with the ascending and descending branches distributed ventrally in the VCN and DCN. Fibers with progressively higher CFs bifurcate and distribute at progressively more dorsal locations.
One consequence of this organization is that the overall length of each auditory nerve fiber remains approximately constant across CFs (Arnesen & Osen, 1978; Fekete et al., 1984). Although apical fibers must traverse a greater distance from their entrance in the spiral lamina to the Schwann–glia border, this length is offset by a shorter distance to the point of bifurcation. In the cat, the total length of the ascending and descending branches after the point of bifurcation is 6–7 mm; the length of the intracochlear axon from the bifurcation to the habenula perforata is also about 6–7 mm. Within the DCN, auditory nerve terminal fields form an anisotropic spatial envelope, with the long axis oriented perpendicular to the ependymal surface and short axis confined to an isofrequency lamina (Ryugo & May, 1993). The orientation of the terminal field shifts systematically with CF, corresponding to the gradual curvature of the DCN.
Owing to technical constraints, most studies of fiber projections have historically analyzed and/or presented data using 2D plots, typically along a parasagittal plane to maximize the information yield across all subdivisions (Fekete et al., 1984; Ryugo & May, 1993). A limitation of such analyses is the difficulty in capturing the undulating variations of isofrequency laminae within all three dimensions. Recently, a quantitative 3D model of frequency representation in the CN was developed for the mouse (Fig. 6.3) that confirmed and extended the 2D projection pattern previously described (Fekete et al., 1984; Ryugo & May, 1993; Muniak et al., 2013). Visualizations of the helical twist of the nerve root and the angular projection of fibers transitioning from PVCN to DCN were also possible (Sando, 1965; Arnesen & Osen, 1978). At the base of the nerve root, low-CF fibers spiral around the outer edge of the nerve bundle along a medial-to-rostral trajectory before bifurcating laterally. Higher-CF fibers enter the nerve root more centrally within the bundle, ultimately bifurcating both at more dorsal and more medial locations than those of lower CFs. Such reconstructions demonstrate that, although the dorsal-ventral axis may be the predominant orientation of fiber distribution with respect to CF, there is also a strong medial-lateral bias that must be considered (Fig. 6.4). The stereotyped primary projection to the CN may be described as forming “C”-shaped laminae that stack vertically upon one another with increasing CFs (Fig. 6.3).
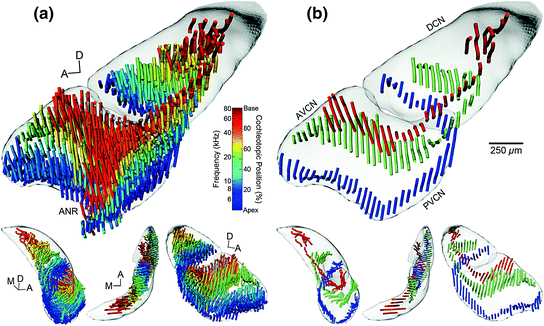
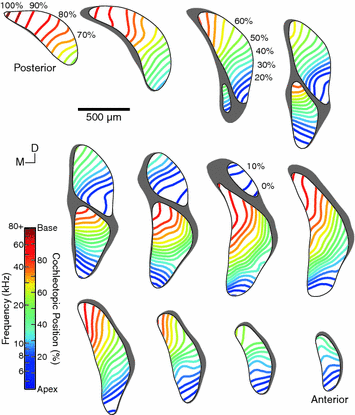
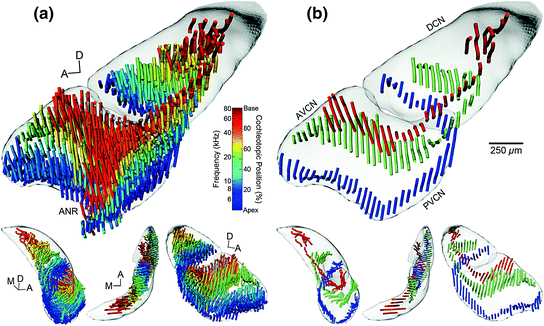
Fig. 6.3
3D tonotopic arrangement of auditory nerve fibers in the CN of the CBA/J mouse. a Reconstructed auditory nerve fiber trajectories from 24 experimental cases are shown after normalization to a template CN. Each tract is represented as a set of “noodles” and shaded according to its cochleotopic origin. An equivalent tonotopic value is calculated based on a place-frequency map of the cochlea. A clear tonotopic trend is evident in all three major subdivisions. DCN and VCN surfaces are also shown and rendered semi-opaque. The top figure is from a medial viewpoint. Lower figures are from alternate viewing angles; L-R: posterior, dorsal, and lateral. In the lower figures, the reconstructed root branch fibers have been removed. b Three cases from a are chosen to illustrate the trajectory of low-, middle-, and high-frequency fiber tracts more clearly. Examples shown correspond to values of 13.5 %/6.4 kHz, 53.0 %/20.5 kHz, and 87.0 %/55.8 kHz. Scale bar equals 250 µm for large figures only (Adapted from Muniak et al., 2013)
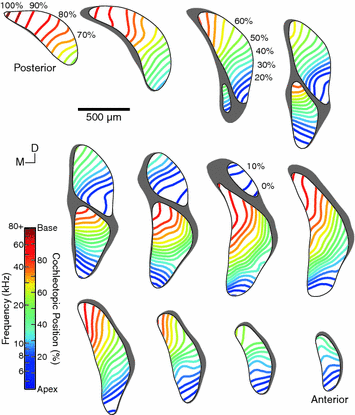
Fig. 6.4
Collection of “virtual slices” through a quantitative model of frequency representation in the CN of the CBA/J mouse. Evenly spaced (150 µm) parallel slices are presented in the coronal plane. Isofrequency lines are rendered within each slice at 5 % cochleotopic intervals, equivalent to 0.25-octave steps. Slices within each row or column are aligned with one another along the orthogonal axis. Frequency representation can be seen to change along both dorsal-ventral and medial-lateral gradients through each subdivision (Adapted from Muniak et al., 2013)
The precise tonotopic projection pattern of auditory nerve fibers provides a solid grounding for observations of orderly frequency tuning in the CN (Bourk et al., 1981; Spirou et al., 1993). Some regions of the nucleus, however, exhibit tuning variations that are explainable on the basis of local perturbations in afferent input. In the first instance, fine-scale frequency organization was described as irregular in the nerve root (Bourk et al., 1981), with high-CF units encountered in locations generally corresponding to low CFs. This circumstance is created by the presence of collaterals arising from the root branch prior to the bifurcation (Fekete et al., 1984). The numbers and lengths of these collaterals can vary widely, but are usually confined to the nerve root region. Fibers with high CFs tend to emit more numerous and extensive collaterals, which can intermingle with low-CF fibers and complicate frequency tuning. Octopus cells, a unique cell type of the PVCN, respond strongly to the onset of sounds or stimulus transients and represent another instance of unconventional tuning (Godfrey et al., 1975; Rhode & Smith, 1986). These cells show sharp tuning near threshold, but exhibit broader tuning 20 dB above threshold (Godfrey et al., 1975). The prominent dendrites of octopus cells intersect a broad swath of descending branch fibers (Osen, 1969), providing a likely substrate for broad tuning. On the other hand, it was noted that descending fibers with CFs greater than 4 kHz emit long collaterals oriented orthogonal to the parent branch, but parallel to octopus cell dendrites (Fekete et al., 1984). The combination of a dendrite intersecting a wide CF swath of auditory nerve terminals and collaterals from a single fiber converging on the same dendritic branch could contribute to narrow tuning near threshold and broad tuning at higher levels.
There has been some question as to whether all frequencies are equally represented in each subdivision of the CN. In the mouse (Muniak et al., 2013) and gerbil (Müller, 1990), reconstructions of tissue volume with respect to CF suggest that proportional volumes of each subdivision are roughly dedicated to equivalent octave ranges (Fig. 6.4). In cats, however, electrode penetrations (Spirou et al., 1993) and fiber reconstructions (Fekete et al., 1984; Ryugo & May, 1993) suggest that greater amounts of tissue in the DCN may be dedicated to mid–high frequencies relative to that in the VCN. Complicating this interpretation, however, is that these data were analyzed with respect to linear distance, rather than tissue volume, which appears not to be uniform (i.e., narrowing cross sections) along the frequency axis. Interestingly, the bat appears to have an expanded representation of the echolocating frequency range (Feng & Vater, 1985). This expansion, however, reflects a similar “fovea” in its cochlear representation (Vater et al., 1985), suggesting that the presence (or absence) of cochlear specializations may be equivalently represented through the projection pattern of auditory nerve fibers.
6.3.2.3 Organization with Respect to Spontaneous Discharge Rate
Hearing involves more than simple analysis of the frequency spectrum. Accordingly, although the predominant organizational feature of auditory nerve projections into the CN is tonotopy, the central projections of type I fibers also exhibit variations with respect to SR, echoing observations in the periphery (Fig. 6.5). Fibers belonging to different SR classes have distinct physiological characteristics, particularly with respect to encoding dynamic range and representing vowel sounds (Sachs & Young, 1979; Evans & Palmer, 1980; May et al., 1996). High-SR fibers with low thresholds may operate best in quiet, whereas low-SR, high-threshold fibers may be optimized for loud and noisy environments. Different groupings of fibers likely contribute to separate roles in acoustic processing and so are expected to differ in their central terminations.
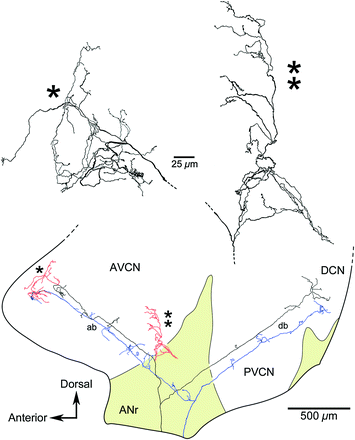
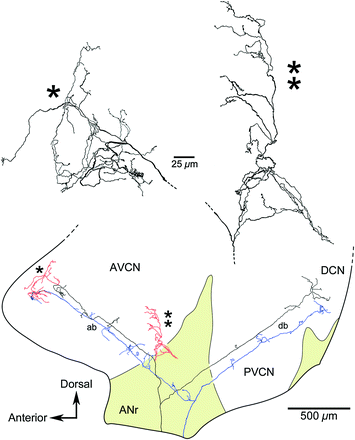
Fig. 6.5
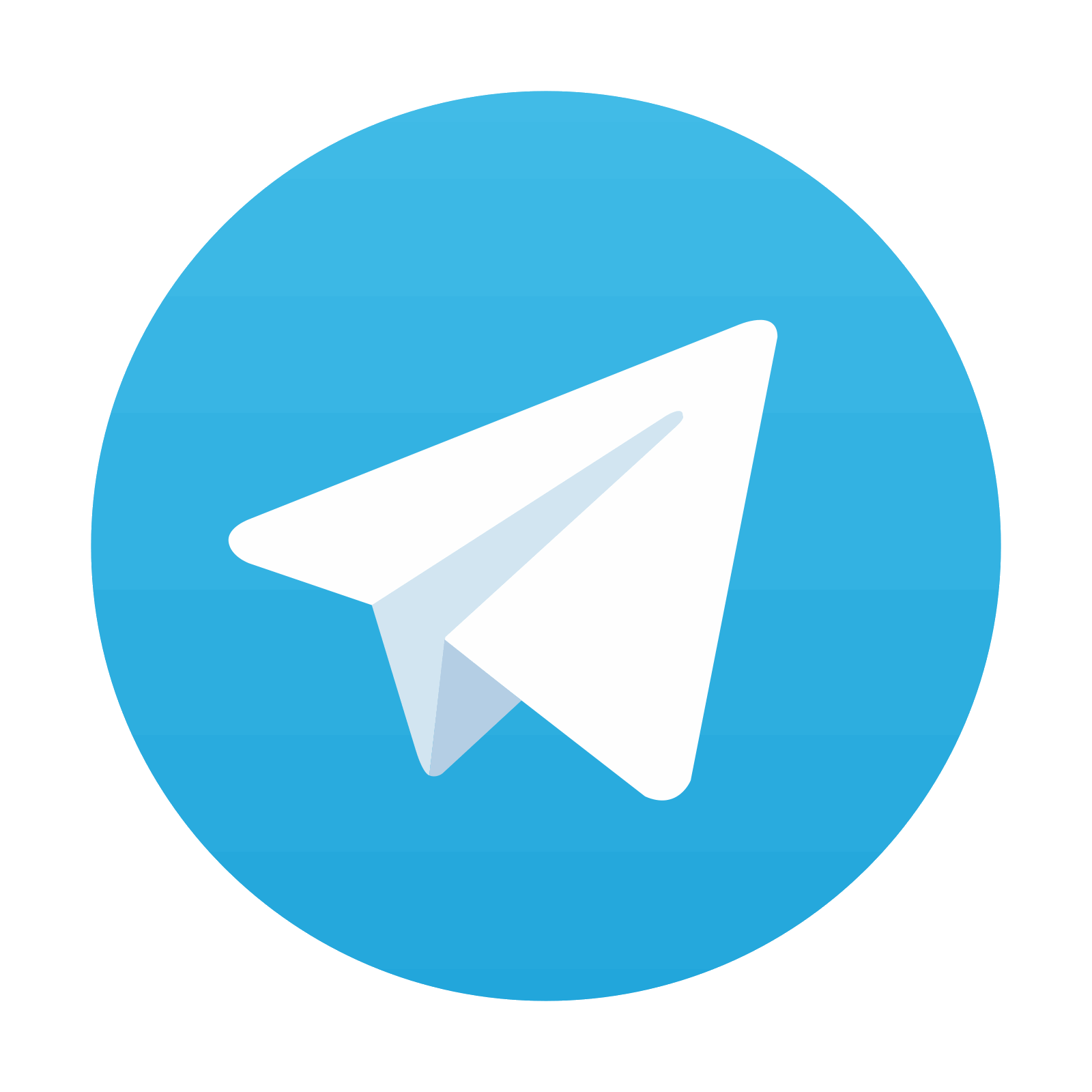
Drawing tube reconstructions of a low-SR auditory nerve fiber (black and red; CF = 3.1 kHz; SR = 0.2 spikes/s; threshold = 26 dB SPL) and a high-SR auditory nerve fiber (blue; CF = 1.2 kHz; SR = 86 spikes/s; threshold = −3 dB SPL), as viewed laterally. The ascending branches take a relatively straight trajectory through the AVCN. Low-SR fibers are distinctive by the collaterals that arborize within the small cell cap (red). Otherwise, the main parts of the ascending and descending branches are similar for the different SR types. Higher magnification drawings are shown for each collateral. One collateral ramifies anterior to the endbulb (*), whereas the other ramifies laterally (**). The collaterals of high threshold, low-SR fibers ramify extensively within the small cell cap and are good candidates for serving as the afferent limb of the high threshold circuit that feeds back to the organ of Corti by way of the olivocochlear system (Ye et al., 2000). ab, ascending branch; ANr, auditory nerve root; AVCN, anteroventral cochlear nucleus; db, descending branch; PVCN, posteroventral cochlear nucleus (Adapted from Fekete et al., 1984; Ryugo, 2008)
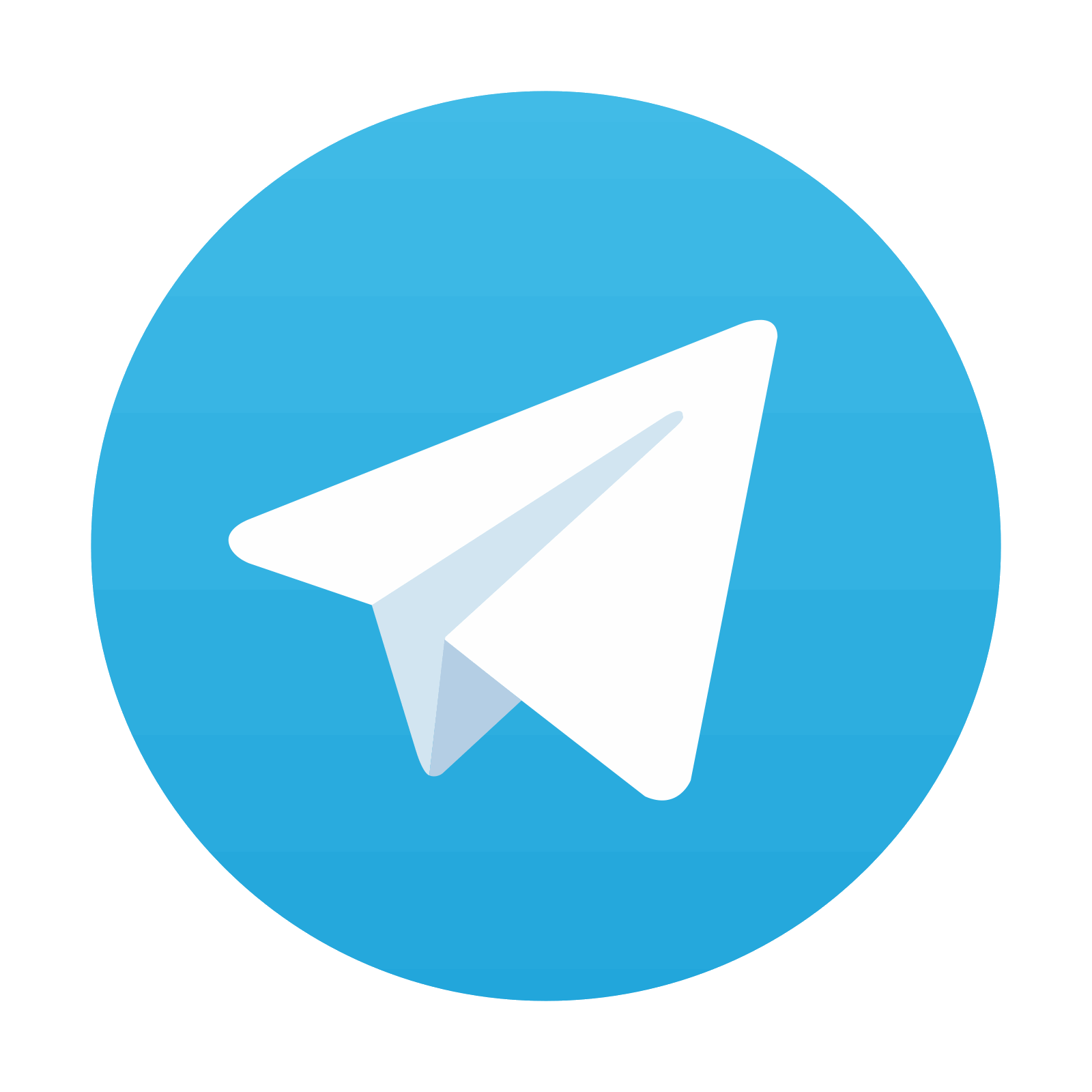
Stay updated, free articles. Join our Telegram channel
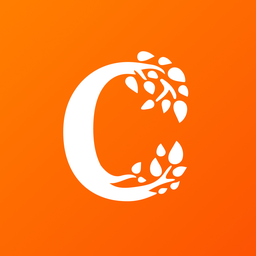
Full access? Get Clinical Tree
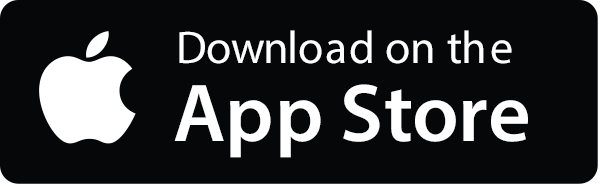
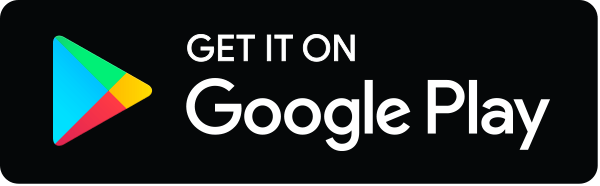
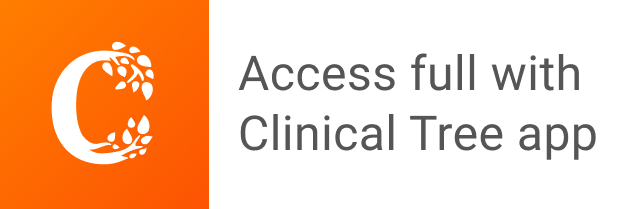