Fig. 70.1
Appearance of atherosclerotic plaque (a) The normal coronary artery is structured into intima (I), media (M), and adventitia (A), which are clearly visualized by OCT. (b) Pathologic intimal thickening is characterized by the thickening of the intimal layer in the absence of a necrotic core. With the progression of atherosclerosis, the border between intima and media becomes diffuse. (c) A fibrous cap atheroma with its typical necrotic core covered by a fibrous cap. (d) A thin-cap fibroatheroma is recognized as a rupture-prone plaque and is characterized by a thin fibrous cap overlying a large necrotic core, usually accompanied by inflammation in the fibrous cap and expansive arterial remodeling
Atherosclerosis is caused by the accumulation of fatty material in the intimal layer of the arteries, accompanied by chronic inflammation. According to the currently accepted view, once monocytes penetrate into the vessel wall, they are taken up by macrophages and become foam cells, which owe their name to their histological appearance. The subsequent signaling cascade eventually results in an advanced lesion with a lipid or necrotic core. The intimal layer that separates the necrotic core from the vessel lumen is usually reinforced by additional collagen and is referred to as the fibrous cap. “Plaque vulnerability”is a term employed to describe the risk of this cap to rupture. Many factors contribute to the mechanical integrity of the fibrous cap, but its thickness is traditionally used as the most obvious indicator of vulnerability, defining lesions with a thin fibrous cap covering a fibroatheroma (TCFA) as most prone to rupture. Figure 70.1b–d displays different presentations of typical atherosclerotic plaques.
A plaque rupture would expose thrombogenic substrates such as von Willebrand factor attracting platelets and fibrin, which results in formation of thrombus. The fate of plaque rupture is determined by three factors: degree of occlusion, duration of occlusion, and the level of preexisting collaterals. When thrombus is nonocclusive, it may become organized leading to rapid progression of atherosclerosis which is clinically silent. When the lumen is partially occluded, the patient develops unstable angina or myocardial infarction without elevation of the ST segment in the electrocardiogram depending on the duration of occlusion and the level of preexisting collaterals. These events are frequently classified as non-ST elevation acute coronary syndromes (NSTE-ACS). When occlusive thrombus persists for more than 20 min, damage to the myocardium becomes irreversible, manifesting in an ST-elevation (STEMI). Another important underlying mechanism for MI or SCD is plaque erosion, particularly in young women and in smokers. It may be responsible for MI or SCD in up to 30–40 % of cases.
Patients with an acute coronary event are usually treated with antithrombotic medications and frequently by percutaneous coronary intervention (PCI). During PCI, a catheter is introduced through the femoral or radial artery to gain access to the coronary arteries. The goal of the procedure is to reopen the flow-limiting lesion, commonly by balloon angioplasty followed by the placement of stents. Balloon angioplasty is performed by inflating a carefully sized balloon inside the identified artery segment to widen the lumen and reestablish sufficient blood flow. The subsequent placement of a coronary stent, a mesh-like structure placed in the artery with a similar inflating balloon, helps to maintain the gained lumen diameter. The initial use of bare-metal stents (BMS) has frequently caused complications due to neointimal growth within the stent, ultimately resulting in restenosis of the vessel. Drug-eluting stents (DES) reduce this restenosis by releasing drugs that block cell proliferation. Hopes are high that bioabsorbable scaffolds currently under development help to further reduce complications. These scaffolds are eventually fully absorbed and provide a more natural compliant structure that is better accepted by the vessel than the previous metallic stents.
The catheterization laboratory is equipped with a fluoroscope to perform angiography by administration of a radiopaque agent. The contrast agent absorbs the X-Ray irradiation and produces a negative contrast on the angiogram and allows the real-time visualization of the vasculature. Angiography is also the primary diagnostic tool to identify the severity of the lesion in the coronary vasculature. The various catheters have radiopaque markers for visualization on the angiogram. A thin (0.0014″) guide wire is first used to gain access to the coronary arteries. A wide guiding catheter is placed at the ostium. The balloon catheters for angioplasty and stenting, and diagnostic imaging modalities such as OCT or intravascular ultra sound (IVUS), are then advanced over the guide wire into the coronary artery.
70.3 State-of-the-Art Intravascular OCT
Several chapters of this book are dedicated to the thorough discussion of the theory and instrumentation of OCT. In view of this, we only give a short description of the basic principles of OCT but highlight some aspects specific to intracoronary imaging.
Optical coherence tomography (OCT) is a high-resolution imaging modality that generates cross-sectional images of tissue microstructure [1]. It utilizes coherence gating to select scattering signals from a defined depth within the tissue by interfering the sample light with a known reference signal. The first-generation OCT technology was based on “time domain” detection (TD-OCT). Near-infrared light with a large spectral bandwidth, and corresponding short coherence length, was focused onto the tissue. Combined with the reference signal, only light from the sample depth corresponding to the reference arm length created an interference signal at the detector. Mechanically scanning the reference arm length sequentially retrieved the axial sample reflectivity profile. Dramatic improvements in sensitivity and imaging speed could be achieved by detection in the Fourier domain. This detection scheme is also known as spectral domain OCT, swept source OCT, or optical frequency domain imaging (OFDI). Optical frequency domain imaging operates with a frequency-swept laser source, changing the wavelength as a function of time over the available spectral range [9, 10]. The reference arm is kept at a stationary position, and the interference between the sample and the reference light now creates a signal whose frequency is proportional to the offset of the signal from the reference arm length. Fourier transformation of this recorded fringe signal recovers the axial sample profile with a single wavelength sweep. Because the signal of a single reflecting element in the sample is reconstructed by combining the detected signal of all wavelengths, a large sensitivity advantage results, which was pointed out independently by three different research groups in 2003 [11–13]. This understanding spurred the development of wavelength-swept laser sources and resulted in an impressive decrease in sweep times, resulting in a dramatic improvement of imaging speeds for OCT. This was vital for intracoronary imaging, as the slow imaging time of previous TD-OCT systems required proximal occlusion or continuous flushing with saline or contrast agent (the same agent used for angiography) to displace the blood. The resulting ischemia and limitation on the injected flushing volume restrained the field of view that could be imaged. The increase in imaging speed was essential to achieve imaging of significant segments of the coronary arteries with injection of only a small volume of clearing agent and make intravascular imaging practical.
Figure 70.2 depicts a schematic of a typical intravascular OFDI system. The wavelength-swept laser source operates with repetition rates of 50–200 kHz centered around 1,300 nm and spanning 120–140 nm, resulting in axial resolution in tissue of 6–8 um [14]. The fiber-based interferometer employs fiber circulators for an efficient signal transduction [15] and shifts the reference signal with an acousto-optic modulator to remove depth degeneracy [16]. The sample arm consists of several meters of single-mode fiber, connecting through the rotary junction to the catheter and providing sufficient manipulation range for its operation in a clinical setting. As a result, the polarization state of the light propagating along the fiber is not preserved, and polarization diverse dual-balanced detection is advantageous. The structural OCT signal is obtained by taking the sum of the squared norm of the reconstructed A-line of each channel. This renders the signal independent of the actual polarization state of the light at the detector and makes the tomogram insensitive to catheter motion.
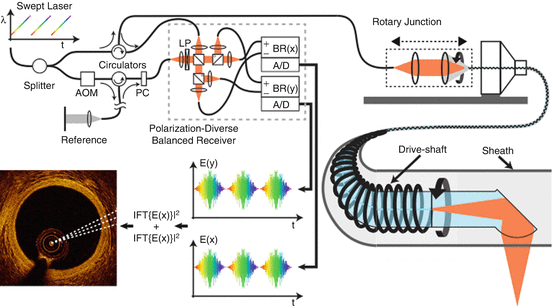
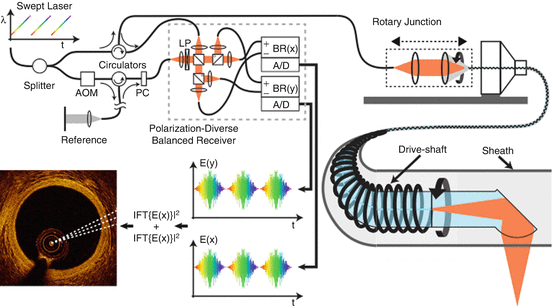
Fig. 70.2
Principle of intravascular optical frequency domain imaging. The fiber-based interferometer is composed of a fiber coupler, splitting the sample and reference light and employs circulators, and acousto-optic modulator (AOM) and polarization controller (PC) to align the reference light with the linear polarizer (LP) at the receiver. BR balanced receiver, A/D analog to digital conversion. The rotary junction couples light to the rotating fiber probe and can pull back the probe within the static sheath. The tomogram is built up by the sequential depth scans, computed from both polarization channels of the receiver
The rotary junction consists of a static collimator on the system side. On the catheter side, a second collimator, which is part of the spinning catheter, couples the light into a single-mode fiber, as shown in Fig. 70.2. The fiber-optic probe is at the distal end of this fiber. The probe is composed either of a graded index fiber, followed by a reflecting prism, or an angle-polished ball lens. The ball lens can be fabricated in an integrative way directly at the fiber tip, by heating a carefully sized coreless fiber that was previously spliced to the guiding single-mode fiber, with a fiber-optic splicer, followed by angle polishing. The fiber connecting the collimator and the fiber probe is protected by a coiled driveshaft which transmits the torque for spinning of the catheter and protects the fiber. The assembly of the fiber probe and the driveshaft containing the sample fiber is contained within a sheath, similar to the ones used in IVUS, with a diameter of 2.6 Fr (0.87 mm). The sheath is fabricated from transparent polyethylene at the distal end to enable the sample light to focus through the sheath material onto the vessel wall. The static sheath protects the vasculature from the rotating probe and also enables the pulling back of the fiber probe within this sheath to perform a helical scan pattern on the lumen surface. The focal length of the probe is adjusted to generate a focus of 25–30 μm intensity full width at half maximum at an offset of ∼2 mm from the sheath surface.
The first report of a system similar to this description was given in 2006 by Yun et al. [17]. Current commercial systems operate in a very similar fashion and allow frame rates, containing 512 A-lines per rotation, of up to 158 frames per second, and pullback speeds as high as 40 mm/s.
70.4 Technology Translation and Commercial Development
The success of intravascular OCT as a research tool and its current adoption to clinical practice was largely facilitated by early commercial translation of this technology. The availability of commercial instruments is important for the widespread access of the clinical community to new technology and has helped to gather many of the insights discussed in the following sections.
LightLab Imaging, Inc. introduced the M2 imaging system in Europe already in 2002. This first-generation TD-OCT instrument operated with 15 frames per second (200 axial scans per frame) using saline flushing and occlusion balloons. The later M3 system was introduced in Japan in 2007 and improved imaging speed to 20 frames per second (240 axial scans per frame). The first commercial frequency domain system, the C7XR TM, was introduced in 2010 and achieved imaging speeds of 100 frames per second (500 axial scans per frame). This represented a more than tenfold increase in imaging speed, enabling higher frame rates and an increased axial scan density, improving significantly the image quality. The higher frame rate also resulted in faster pullback speeds, which enabled occlusion-free imaging using injection of contrast to displace the blood. (C8 OPTIS system became available in the United States. We will have a new system within a couple of weeks. Do you want to add it?)
Recently, Terumo Inc. (Tokyo, Japan) has entered the market for intravascular OCT imaging by introducing the Lunawave OFDI system, first in Europe in 2012, and then followed by Japan in 2013. It provides imaging at 158 frames per second and pullback speeds of up to 40 mm/s over a length of 150 mm. These improvements in imaging speeds and system performances facilitate the clinical adoption of intravascular OCT and promise to enable a wide range of clinical studies.
Besides the application for intracoronary imaging, Avinger (Redwood City, CA) has launched an intravascular crossing device with OCT image guidance to corkscrew through chronic total occlusions (CTO) in the peripheral vasculature. From the OCT signal, the clinician can identify the vessel wall and guide the crossing catheter through the lesion without cutting through the vessel wall.
70.5 Ex Vivo Validation Studies
Besides attempting the challenge of in vivo measurements, early studies thoroughly investigated the architectural features that can be visualized with OCT and validated these observations with histology. From a correlation study of 357 atherosclerotic arterial segments from 90 human cadavers, we established the OCT definition of fibrous, fibrocalcific, and lipid-rich plaques (Fig. 70.3). A high sensitivity and specificity (90–98 %) of these criteria and high reproducibility between two observers was demonstrated [18]. Besides plaque characterization, different types of intraluminal thrombus (red thrombus and white thrombus) were reported [19]. Figure 70.4a, b displays an example of a red and a white thrombus. Further, we demonstrated the capability of OCT to visualize macrophage accumulation [20]. The infiltration and accumulation of macrophages is an essential component of vulnerable plaques. The resolution of OCT is insufficient to accurately resolve these macrophages. However, due to their significant uptake of lipids, they approach a size similar to the axial resolution of OCT and comprise a structure with a relatively heterogeneous index of refraction. Normal or fibrous intimal tissue consists of many scattering agents spaced on a sub-resolution scale and results in a homogenously scattering layer with fully developed speckle. The signal from the macrophages, however, is composed of more discrete scattering centers of the macrophage wall and the extracellular matrix but with only little contribution from the intracellular structures. The resulting intensity pattern features a contrast that is sufficiently different from the normal intimal tissue to enable clear identification of regions with macrophages. Frequently, the macrophages also cast a shadow on the underlying tissue. Figure 70.4c shows an example of macrophage accumulation. Using an adequately normalized standard deviation to quantify macrophage accumulation from the OCT intensity signal within regions of interest in the fibrous cap, a close correlation with the percent area of CD68 positive cells determined by histology was demonstrated [20]. Although the potential of quantifying macrophages by OCT has attracted a significant amount of attention, it is still awaiting more widespread application and should be validated in subsequent studies and with more recent imaging devices.
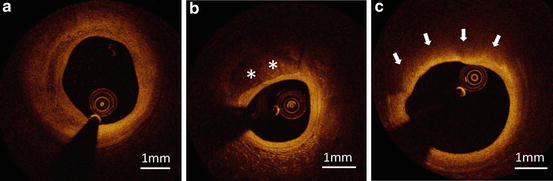
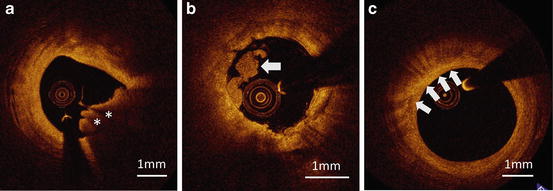
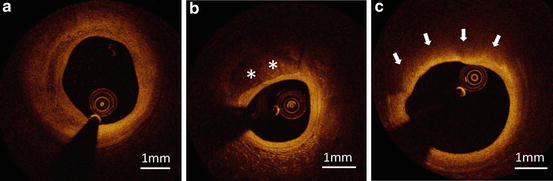
Fig. 70.3
(a) Fibrous plaque is characterized by homogeneous, signal-rich regions. (b) Fibrocalcific plaques are identified by well-delineated, signal-poor regions with sharp borders (asterisks). (c) Lipid is characterized by signal-poor regions with diffuse borders (arrows)
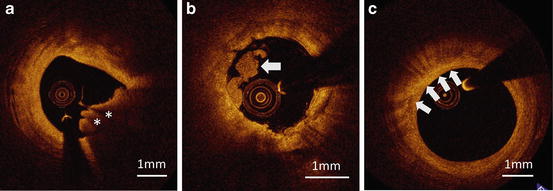
Fig. 70.4
(a) Red thrombus is defined as a mass protruding to the lumen, showing high signal intensity on the surface with rapid signal attenuation along depth (asterisks). (b) White thrombus is a protruding mass showing a lower backscattering signal with low signal attenuation (arrow). (c) Macrophage accumulation is characterized by increased signal intensity within the plaque, accompanied by heterogeneous backward shadows (arrows)
The superior spatial resolution of OCT is crucial for the measurement of fibrous cap thickness and identification of TCFA. In pathology, TCFA is defined as an advanced atherosclerotic plaque showing a large necrotic core with infiltration of inflammatory cells and a thin overlaying fibrous cap. In an ex vivo histology study, an excellent correlation between OCT and histology-measured fibrous cap thickness (r = 0.90) was demonstrated, based on 35 lipid-rich plaques from 102 coronary segments in 38 human cadavers [21]. This makes OCT the only in vivo imaging modality that has been validated with histology to be able to accurately measure fibrous cap thickness.
70.6 First Intravascular Imaging
After promising results from such ex vivo studies and first in vivo animal studies [5, 6], our group at MGH performed the first in-patient study in 2002 [7]. The OCT instrument was a prototype developed at MGH, and the OCT catheter used in this study was constructed using modified 3.2 Fr IVUS catheters (Fig. 70.5). The fiber probe had a miniature gradient index lens and a microprism to focus the optical beam onto the lumen. The bulk system rotary junction allowed a catheter rotation speed of up to 8 rotations per second. The limited image acquisition rate of this TD-OCT system required 8–10 cc of saline to be intermittently flushed through the guiding catheter to displace the blood and clear the view. Both IVUS and intravascular OCT were performed on 17 lesions from ten patients. The comparison demonstrated the potentially superior diagnostic ability of OCT over IVUS for the detection of various plaque components [7].
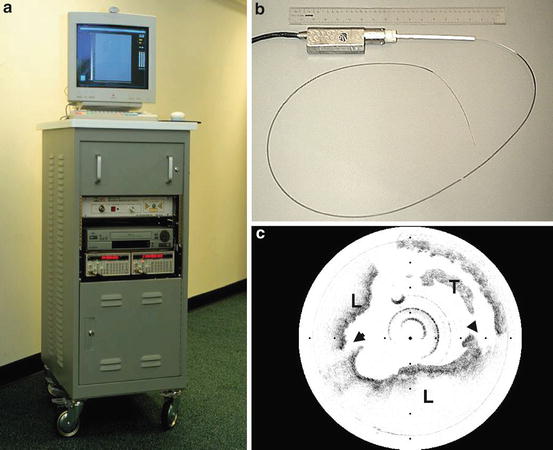
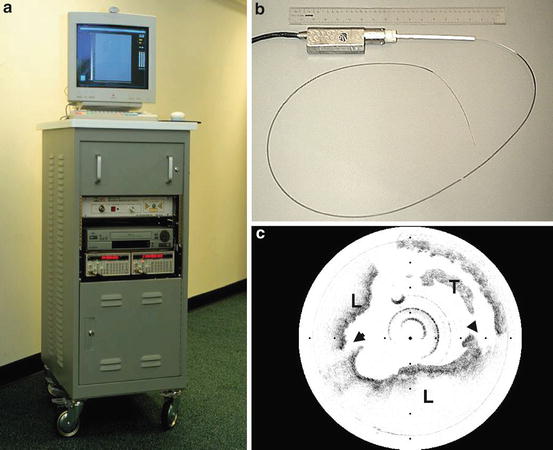
Fig. 70.5
(a) Prototype OCT imaging system and (b) OCT catheter, used for the first human study. (c) OCT visualization of ruptured plaque (arrowheads: site of rupture; T thrombus, L lipid)
In a subsequent study, we analyzed the culprit lesions for a broader range of clinical presentations, including patients with STEMI, NSTE-ACS, and stable angina pectoris (SAP) [22]. Out of 69 patients enrolled in the study, sufficient image quality was obtained in 57 patients (20 STEMI, 20 NSTE-ACS, and 17 SAP). TCFA, defined as a plaque with less than 65 μm of fibrous cap thickness and more than 90° of lipid, was more frequently observed in STEMI and NSTE-ACS patients as compared to SAP patients (72 %, 50 %, and 20 %, respectively, p = 0.012). Further, the measured fibrous cap thickness was thinner in STEMI and NSTE-ACS patients than in SAP (47.0 μm, 53.8 μm, and 102.6 μm, respectively, p = 0.034). This was the first study that demonstrated significant differences in plaque characteristics in vivo depending on the clinical presentation. Moreover, these first in-man studies confirmed the safety and feasibility of intracoronary OCT.
Whereas TD-OCT has been widely used as a research tool for the study of atherosclerosis, its slow imaging speed either required the use of occlusion balloons, which made the imaging procedure more cumbersome and uncomfortable for the patient, or only permitted the imaging of relatively small sections of the arteries. The advent of the faster spectral domain systems has largely overcome these limitations. We reported the first human in vivo imaging with a spectral domain system in 2008 [23]. The commercial availability of spectral domain systems since 2010 made these advantages also accessible to the wider clinical community and has aided in the ongoing clinical adoption of intravascular OCT.
70.7 Assessment of Coronary Atherosclerosis
In this section we are describing important insights regarding plaque morphology and the pathogenesis of ACS gained by the use of OCT in patients. Previously, clinical imaging modalities such as IVUS, angiography, or computed tomography (CT) lacked sufficient resolution to assess crucial parameters of plaque characteristics. The unprecedented spatial resolution by OCT provided access to decisive plaque features that were correlated extensively with clinical presentation and contributed to clarifying the pathophysiology of coronary atherosclerosis.
Estimating macrophage density by computing the normalized standard deviation in the OCT signal of fibrous and lipid-rich plaques was found to be significantly higher in ACS patients compared to SAP patients [24]. This matches well with the understanding that macrophage accumulation weakens the fibrous cap and hence makes it more prone to rupture [25].
Patients presenting with ACS are known to have lesions with expansive arterial remodeling. The remodeling index is expressed quantitatively by the ratio of the cross-sectional area of the external elastic lamina at the lesion site, with a reference point presenting a more normal vessel appearance. Positive remodeling corresponds to an increased external elastic lamina cross section at the lesion with respect to the reference. Negative remodeling, although less frequent, represents a smaller vessel diameter at the lesion site than at the proximal reference. The remodeling index is a parameter established from IVUS and requires imaging through the entire thickness of the lesion. As such, it is beyond the reach of OCT. In a study where we sequentially imaged with IVUS and OCT [26], we found that TCFA was more frequently observed in lesions with positive remodeling than in those with intermediate or negative remodeling (80 %, 38.5 %, and 5.6 %, respectively, p <0.001). Likewise, the number of lipid quadrants of the lesions correlated positively with the remodeling index [26].
Another factor that is considered to contribute to plaque stability is intra-plaque neovascularization. These blood vessels are shown in OCT images as vesicular structures, or micro-channels, within the plaque. Neovascularization visualized with OCT has been associated with a thin fibrous cap [27], poor responsiveness to statin therapy [28], and plaque progression [29].
The spatial resolution of OCT also enables to study the detailed morphology of fibrous cap disruptions. Tanaka et al. [30] analyzed plaque disruption in 43 patients and compared the features with the exertion level of the patients at the onset of ACS. They found that shoulder-type rupture was more frequent in patients on exertion than those at rest. In another study investigating plaque rupture, STEMI patients were found to suffer more ruptures than patients presenting with NSTE-ACS (70 % vs. 47 %, p = 0.033), and cap disruption tended to be directed against the flow in STEMI patients [31].
Cap thickness is generally considered one of the most important predictors of plaque rupture. In a pathological study the minimal fibrous cap thickness was measured in the histological sections of 41 ruptured plaques that had caused sudden cardiac death [32]. The 95th percentile value was found at 65 μm and is the widely accepted threshold for a rupture-prone plaque. Yonetsu et al. [33] performed an assessment of the minimal fibrous cap thickness in an OCT study comparing ruptured with non-ruptured lipid-rich plaques in vivo. A fibrous cap thickness <80 μm was found as the 95th percentile value, which is consistent with expected tissue shrinkage of 10–20 % during histopathologic processing.
Besides the culprit lesion, non-culprit or remote lesions were also studied by OCT. Non-culprit lesions of patients with ACS presented more vulnerable features than remote lesions of patients with SAP: greater lipid amount, thinner fibrous cap thickness, and more neovascularization close to the lumen [34]. These results suggested that ACS is a systemic, pan-vascular disease rather than a focal phenomenon of the coronary arteries.
With its ability to precisely assess relevant plaque characteristics, OCT could prove to be ideally suited to evaluate the pathophysiology of ACS [35]. Ruptured plaque is recognized as the primary cause of ACS. However, pathological studies showed that plaque erosion and calcified nodules account for approximately 20 % of sudden cardiac deaths and 25–40 % of acute coronary thrombosis [36–38]. Plaque erosion is defined in pathology as the formation of a thrombus on the surface of a non-ruptured plaque with denudation of the endothelial layer [36]. And a calcified nodule is defined as a calcified plaque with dysfunctional or entirely lacking endothelial layer, resulting in a protruding calcium nodule without fibrous cap [36]. The resolution of OCT is insufficient to detect the presence or absence of the endothelial layer, and the limited image penetration makes it difficult to detect a fibrous plaque behind a massive thrombus [39]. This makes it difficult to apply the pathological definitions directly to OCT. Instead, we proposed an alternative OCT definition of plaque erosion and calcified nodules in collaboration with pathologists. Our definition classifies the culprit lesions of ACS into plaque rupture, calcified nodules, definite erosion, probable erosion, and an unclassified (other) group [40]. Figure 70.6 displays an example of definite erosion and calcified nodules. Using this definition, we analyzed a total of 126 ACS culprit lesions with OCT and found that 55 (44 %) showed plaque rupture, 39 (23 %) OCT-identified erosion (23 definite erosions and 16 probable erosions), and 10 (8 %) OCT-derived calcified nodules. Erosion was found more frequently in younger patients and NSTE-ACS rather than STEMI. This corresponded well with data from pathology, suggesting that the OCT-derived definition of erosion matches well the pathological definition. Taking into account the different mechanisms and patient backgrounds leading to ACS, it would be important to consider different therapeutic strategies for these patients. The current treatment strategy for ACS patients, and especially STEMI, presumes that coronary thrombosis occurs as an effect of plaque rupture. However, cases caused by erosion or calcified nodules could be treated with antithrombotic agents instead of stenting, because these lesions theoretically have less occlusive plaque underneath the thrombus and thus could be dissolved with antithrombotic therapy.