Fig. 18.1
Slit lamp photo of (a) age-related cortical cataract; (b) age-related nuclear cataract
Cortical Cataracts Cortical spokes (wedge shaped opacities) form in the periphery of the lens with the pointed end of these spokes oriented toward the center (Fig. 18.1). Changes in the ionic composition of the lens cortex with hydration of the lens fibers lead to the formation of cortical spokes [14].
Posterior Subcapsular Cataracts (PSC) PSCs, which are located in the posterior cortical layer may later turn into a granular and plaque-like opacity. PSCs are associated with posterior migration and enlargement of lens epithelial cells. They can occur due to aging as well as trauma, systemic or topical steroids, inflammation in the eye (uveitis), and exposure to ionizing radiation [14].
18.2.3 Etiology and Risk Factors
There are limited ways of repair and regeneration for a lens, and, thus, it may lose its transparency due to many different factors.
Aging Aging is a very common cause of cataract formation. The pathogenesis is multifactorial. The nucleus of a lens becomes compressed and hardened by the addition of cortical fibers. During aging, crystalline lens proteins are changed by chemical modification and they aggregate into high molecular weight proteins. The aggregation of lens proteins reduces transparency and causes a yellowish pigmentation. Increased concentration of sodium and calcium together with decreased potassium and GSH are other age-related changes [14].
Physical and Environmental Factors Physical factors like trauma and electric shock can lead to cataract formation. A lens may react to penetrating and non-penetrating chemical injuries and intralenticular foreign bodies in different ways. If the capsule of the lens is intact, a cataract forms; if the capsule is ruptured, water enters into the lens and a mature cataract forms [15]. If the lens is penetrated by a foreign object, the capsule may heal and a localized capsular opacity may form. Unless a seal forms, a severe inflammatory response may occur in the eye. A lens can also be damaged by lightening or accident. Voltages as low as 220 V may cause cataract formation [15].
Exposure to metals and cigarette smoke may also lead to cataract formation. Copper, for example, may form deposits in the eye from eye drops, from a copper-containing foreign body, or due to Wilson’s disease (autosomal recessive deficiency of ceruloplasmin). These patients develop a typical sunflower cataract [16]. Iron forms deposits in the lens epithelium and iris leading to cataract and iris heterochromia [15], whereas gold forms deposits on the lens capsule and epithelium causing an anterior capsular cataract [15]. Cigarette smoke affects the eye mainly through ischemic or oxidative mechanisms. Both cataract development and age-related macular degeneration are directly accelerated by smoking [17].
The lens is very sensitive to radiation, including, ionizing, nonionizing, ultraviolet, and even microwave radiations. Although there is a long period of latency before the clinical appearance of a cataract, younger patients are more susceptible as they have more actively growing lens cells [14]. Ionization of water and the formation of free radicals, as well as hydration of electrons, damage cell membranes causing transcriptional errors and altering protein synthesis. The lens equatorial fibers that have high mitotic rates are affected and posterior subcapsular opacities form. Nonionizing radiation, i.e., infrared radiation, causes cataracts in glassblowers and furnace workers. A localized temperature rise of the iris pigment epithelium causes exfoliation of the anterior lens capsule [15]. UV radiations are also known to generate free radicals, which generally causes nuclear cataract. Interestingly, microwave radiation has been shown to cause cataracts in laboratory animals.
Medicinal Drugs Use of a number of medicinal drugs have been associated with cataract formation, including corticosteroids, phenothiazines, anticholinesterases, mitotic drugs, busulfan, amiodarone, and chloroquine. Systemic, topical, subconjunctival, and nasal steroid sprays can all cause cataracts, predominantly posterior subcapsular cataracts [14]. Chlorpromazine, a phenothiazine can generate free radicals leading to cataract formation. Initially, yellow-brown granules form under the anterior capsule and develop into stellate opacities [15]. Anticholinesterases, which were used for the treatment of chronic angle closure glaucoma, were shown to cause anterior subcapsular vacuoles. Busulfan, which is used in the treatment of leukemia may lead to posterior subcapsular cataracts, whereas amiodarone, which is used for cardiac arrhythmias, is known to cause visually insignificant anterior subcapsular opacities as well as corneal deposits [18]. Chloroquine, an antimalarial drug, causes white, flake-like posterior subcapsular cataracts [15].
Ocular Disorders Ocular disorders are also a risk factor for developing cataracts. Retinitis pigmentosa (RP), which is an inherited retinal dystrophy caused by the loss of photoreceptors, is characterized by typical posterior subcapsular cataracts [19]. Patients with gyrate atrophy, an autosomal recessive slowly progressive chorioretinal dystrophy, present with nyctalopia and limited peripheral visual fields. They also develop cystoid macular edema and cataracts [20]. Degenerative myopia is a major risk factor for posterior subcapsular, cortical, and nuclear cataracts [21]. Ciliary body tumors may also cause cataracts. These tumors may not be recognized behind a cataractous lens, which may lead to a delayed diagnosis. Ocular ischemia, another risk factor for cataract development, is characterized by vision loss, orbital pain, and changes of the visual field. Both anterior and posterior segment signs may be present. Anterior segment signs include iris neovascularization and secondary neovascular glaucoma, iridocyclitis, asymmetric cataracts, iris atrophy, and sluggish reaction to light [22]. Cataracts and glaucoma are the main complications of viral anterior uveitis due to the herpes simplex virus/varicella zoster virus (HSV/VZV), cytomegalovirus (CMV), and rubella [23]. Both intraocular inflammation and steroid therapy for inflammation can cause cataracts to form.
About a third of congenital cataracts is hereditary and is not associated with any systemic or metabolic disorder. Some of these may be associated with trisomy 13, 18, 21 and cri du chat syndrome [15]. The incidence of infantile cataracts is about 0.4 % of newborns [15]. These are important because, if unrecognized, they may lead to amblyopia, strabismus, and nystagmus. The causes of infantile cataracts may be maternal infections like rubella, systemic diseases, hereditary disorders, and local ocular disease.
Nutritional deficiencies have been demonstrated to cause cataracts in animal models; however, this has not been confirmed in humans [14]. One of the major risk factors for cataract formation is any type of intraocular surgery. Although there are numerous systemic disorders that are known to cause cataracts, they are beyond the scope of this chapter and, therefore, are not discussed here.
18.3 Oxidative Stress and Cataract Formation
18.3.1 Oxidative Stress
Oxidative stress, the loss of balance between the antioxidant defense and oxidant production in cells, has been implicated in the etiology and pathogenesis of cataracts and other eye disorders. Reactive oxygen species (ROS), a byproduct of cellular respiration and metabolism, are known to play a dual role as both deleterious and beneficial species. They are important physiologically in cellular signaling pathways and as defense against infectious agents and induction of mitogenic responses [24–28]. Their production is tightly regulated by various enzymes; however, overproduction of ROS, either through photooxidation or metabolic processes, can damage cell structures, including DNA, lipids, and proteins [25, 26, 29]. Balance between the dual roles of ROS is crucial and is achieved by redox regulation.
ROS can be generated endogenously or exogenously from the environment. The endogenous sources include mitochondria, peroxisomes, lipooxygenases, NADPH oxidase, and cytochrome P450. Leakage of electrons from complexes I and III of the electron transport chain in mitochondria is the major source of ROS in cells [30]. In addition to producing ROS, mitochondria are also a target for ROS which, in turn, reduces mitochondrial efficiency and leads to the generation of more ROS, a vicious self-destructive cycle. Exogenous sources include ultraviolet light, ionizing radiation, smoke, chemotherapeutics, inflammatory cytokines, environmental toxins, and hyperoxic environments [31, 32]. However, due to its anatomical locale, the lens is constantly exposed to light and radiation and is more vulnerable to these exogenous sources of ROS.
The superoxide radical (O2 •−), hydroxyl radical (OH•), singlet oxygen (1O2), and hydrogen peroxide (H2O2) are the most commonly formed ROS in a cell. Other oxygen-derived radicals like peroxyl (ROO•) radical and protonated superoxide (HO2 •) are also formed in living systems. Although, there are numerous types of ROS, the most reactive and damaging species are those that are free radicals due to the presence of unpaired valence electrons. The O2 •− is the primary ROS which, in turn, generates secondary ROS species by dismutation to H2O2 and eventually OH•. H2O2 is a mild oxidizing agent which is relatively stable. However, the OH• formed by Haber-Weiss reaction is very reactive with a short in vivo half-life of approximately 10−9 s [33]. When OH• is produced, it reacts with macromolecules in its proximity resulting in lipid peroxidation as well as oxidation of proteins and nucleic acids.
Oxidation is the hallmark of many types of cataracts and plays a fundamental pathogenic role in their formation. Clinical and experimental studies have provided substantial evidence that ROS and the resulting oxidative damage are the key contributing factors to the development of a variety of cataracts [34–40]. Significant physiological and biochemical changes indicating oxidative damage in cataractous lenses have been identified by comparisons of the lipid compositions, protein and DNA modifications, and concentrations of antioxidants in cataractous and clear lenses [40–42]. Photooxidation [43, 44], xenobiotics [45–48], and impaired enzymatic and nonenzymatic defenses in the eye lens are some of the factors that contribute to oxidative stress [49–53].
In addition, hyperbaric oxygen therapy and oxidative stress produced similar biochemical changes and promoted cataract formation [54, 55], which substantiates the hypothesis that oxidative stress is involved in the pathogenesis of cataracts. A direct link between excessive oxygen exposure and nuclear cataracts has also been documented in some studies [55–58]. Elevated levels of O2 •−, H2O2, OH•, nitric oxide (NO), and glutathione disulfide (GSSG), peroxides, depletion of GSH, and oxidation of methionine and cysteine moieties in cataractous lenses, all indicate the involvement of ROS and RNS in cataract formation [59–65].
Under normal physiological conditions, the metabolically active cells of the lens have very high levels of antioxidants, like GSH and a series of defense mechanisms that protect it from the harmful effects of oxidations. These systems provide ample protection for the tissues against oxidation and various chemical modifications. However, under unusual stress, these defenses may not be sufficient to deter oxidative damage and visual impairment.
18.3.2 Oxidative Damage
Free radicals are generated by self-propagating chain reactions that involve initiation, propagation, and termination of free radicals. However, uncontrolled generation of free radicals results in oxidation of macromolecules and, eventually, in the disruption of function and integrity of the cell. The lens is subjected to oxidative stress throughout life, especially because of its anatomical locale, and is one of the leading causes of cataracts, especially maturity-onset cataracts [66–68]. Oxidation of proteins, lipids, and DNA has been implicated in cataractogenesis [69–73]. Furthermore, damaged macromolecules in a mature lens accumulate in the inner region as only the peripheral tissue is metabolically active [67, 68, 74–76]. This limited ability of fiber cells to repair themselves makes the lens susceptible to damage that can lead to the accumulation of protein, lipid, and DNA modifications in nucleated lens cells [67].
Protein Modifications A lens has an unusually high concentration of protein, about 35 % of its wet weight, although it may be as high as 50 % in the nuclear region. A majority of these proteins are crystallins, which are structural proteins with a high level of thiol groups in a reduced state [74]. α-crystallins help maintain the proper folded state of β-crystallins and γ-crystallins, which is crucial for maintenance of lens transparency by acting as a molecular chaperone and binding to β-crystallins and γ-crystallins [77, 78]. Under physiological conditions, the lens utilizes its various antioxidant defenses to effectively protect itself and maintain a reduced state of thiols, which is essential for retaining clarity of the lens. However, under oxidative stress, excess ROS can react with several amino acid residues resulting either in modified less active enzymes or in denatured nonfunctioning proteins [79, 80]. Sulfur-containing residues are the most susceptible ones to ROS attack. In addition, depletion of GSH leads to S-thiolation of lens proteins by oxidized nonprotein thiols to form protein-S-S-glutathione (PSSG), which is one of the initial events leading to conformational changes, including unfolding of crystallins and other modifications [81, 82]. These events are then followed by oxidation of methionines and cysteines and, subsequently, to the formation of protein-S-S-cysteine (PSSC) and protein–protein disulfide (PSSP). Accumulation of the resulting high molecular weight insoluble aggregates results in cataracts [83, 84]. A progressive loss of protein sulfhydryl groups, together with increased oxidation of methionine residues, is observed with worsening cataracts until >90 % of the cysteine and about 50 % of the methionine residues are oxidized in the advanced stages [84]. The amounts of PSSG and PSSC increase with age and are inversely proportional to the free GSH pool [85]. Elevated levels of PSSG and PSSC have been reported in hyperbaric oxygen-induced nuclear cataracts in old guinea pigs [86]. Oxidative stress induces formation of PSSG or PSSC, irrespective of the type of stress [87, 88]. A positive correlation between the level of protein thiolation and nuclear opacity has also been reported [89].
Oxidative damage to protein may lead to structural and functional changes, including conformational changes leading to inhibition of enzymatic and binding activities, fragmentation, denaturation, aggregation, altered gene regulation and expression, and modulation of cell signaling [90]. Crystallins and other proteins in lens fiber do not regenerate during the lifetime of the lens and, therefore, formation of inter- and intra-molecular conjugates, resulting from oxidative damage, will result in aggregation and accumulation of these damaged proteins and lead to cataract formation.
Lipid Peroxidation Oxidative stress, resulting from overproduction of ROS together with impaired enzymatic and nonenzymatic defenses of the lens, is believed to initiate lipid peroxidation (LPO), a causative factor in the mechanism of cataractogenesis. LPO is a widely accepted mechanism of cellular injury and lipid peroxides are used as markers of oxidative damage in cells and tissues. The LPO chain reaction is initiated by the formation of a lipid radical through hydrogen abstraction from a methylene carbon on a polyunsaturated fatty acid (PUFA) by an OH•. The resulting carbon-centered lipid radical rearranges and readily reacts with molecular oxygen in an aerobic environment to form a ROO•. These radicals are unstable and rearrange through cyclization to form endoperoxides and, eventually, a complex series of compounds, including a reactive carbonyl compound, of which malondialdehyde (MDA) is the most abundant [91, 92]. ROO• can also abstract hydrogen from the side chains of neighboring PUFAs, and form hydroperoxides. Hydroperoxides are toxic and may react with transition metals to form stable aldehydes like MDA that damage the cell membranes. This results in the propagation of the chain reaction which subsequently damages cytosolic, as well as membrane proteins [47, 51, 93–95]. LPO has been reported to cause changes in membrane function, including increased permeability [96–99], inactivation of Na+ / K+-ATPase and Ca2+-ATPase [100, 101], and increased membrane rigidity. Furthermore, they may damage the lens by cross-linking with proteins and nucleic acids and also by disturbing the cellular thiol-redox status by depleting the total GSH content, which may lead to formation of protein aggregates with low solubility in the lens matter [102, 103]. Accumulation of these LPO products has been observed in the aqueous humor (AH) samples as well as in the lens membranes of some patients with cataracts [51, 104, 105].
DNA Damage ROS are reported to be mutagenic and can oxidatively damage DNA [106–108] by causing strand breaks and modifications of bases, including the oxidation of guanine residues into 8-hydroxy 2-deoxyguanosine (8-OHdG). Extensive DNA damage has been reported in the epithelial cells of cataractous lens [71, 106]. Mutations in the epithelial cells may lead to loss of transparency as they differentiate into fiber cells. The 8-OHdG is the most extensively studied DNA lesion and is a sensitive biomarker of oxidative DNA damage which can aid in the early diagnosis and treatment of patients with eye disorders [109, 110]. The extent of DNA damage and rate of repair depend on the nature of DNA-damaging agents [107].
18.4 Antioxidant Defense Network in the Lens
Because lenses are constantly exposed to light, they are extremely vulnerable to oxidative damage. However, they have several mechanisms that provide protection against oxidative stress and help maintain their redox state. Several layers of defense are employed to help prevent damage and to repair, recover, or degrade damaged molecules. These include nonenzymatic (e.g., GSH, ascorbic acid, vitamin E, and carotenoids) as well as enzymatic (e.g., superoxide dismutase, glutathione peroxidase, and catalase) systems. However, both of these defense networks are compromised with age and in cataracts [50, 83, 111–115]. In addition, lens cells contain enzymes that can repair damaged lens proteins.
18.4.1 Non Enzymatic Defense
Glutathione (γ-glutamylcysteinylglycine; GSH) and ascorbate serve as the primary nonenzymatic defenses against oxidative stress in the lens. In addition, vitamin E and carotenoids also provide some protection against oxidative stress.
Glutathione GSH is a tripeptide containing amino acids, glutamate, cysteine, and glycine (Fig. 18.2). An unusual γ-linkage between glutamate and cysteine prevents GSH from being readily hydrolyzed resulting in considerable stability of GSH within the cell. GSH is synthesized by the action of the two enzymatic processes. First, glutamate and cysteine are linked together through a gamma peptide bond by γ-glutamylcysteine synthetase (γ-GCS) to form γ-glutamylcysteine. In the second reaction, γ-glutamylcysteine reacts with glycine to form GSH by the enzyme glutathione synthetase (GS). GSH synthesis is primarily dependent on GCS activity, cysteine availability, and GSH feedback mechanisms.
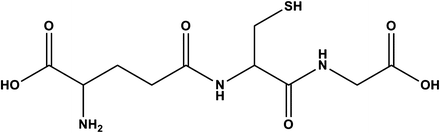
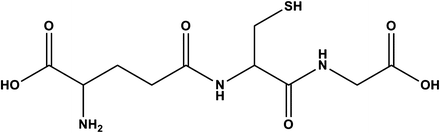
Fig. 18.2
Structure of glutathione (GSH)
GSH is the major thiol antioxidant (2–4 mM) and redox buffer in the lens [89]. It is the first line of defense against exogenous and endogenous ROS. Maintenance of redox homeostasis is crucial for lens transparency and the lens possesses both biosynthetic and regenerating systems for GSH. There is a concentration gradient of GSH with highest levels in peripheral lens fiber cells, where GSH is synthesized and reduced [63] from where it reaches the lens nucleus by diffusion from the surface cells [116]. GSH in the nucleus maintains the redox state of critical protein sulfhydryls required for DNA repair and expression. Oxidation of GSH results in formation of GSSG and decreases the ratio of GSH/GSSG, an important parameter of oxidative stress.
GSH maintains cellular redox homeostasis and protects against oxidative stress by [117]: (1) acting as a cofactor for key antioxidant enzymes like glutathione peroxidase (GPx), glutathione transferase, (2) participating in amino acid transport through the plasma membrane, (3) scavenging OH• radical and singlet oxygen directly, (4) detoxifying H2O2 and lipid peroxides by the catalytic action of glutathione peroxidase, and (5) regenerating Vitamins C and E.
GSH maintains the critical thiol groups in proteins involved in normal lens epithelial functions, such as Na+/K+-ATPase, certain cytoskeletal proteins, proteins associated with normal membrane permeability, and proteins involved in signaling such as receptors, protein kinases, and some transcription factors in a reduced state. Functions of these critical proteins can be altered by formation of mixed disulfides under oxidative stress when the GSSG content increases.
Decreased levels of GSH have been reported in a majority of the experimental cataracts [59, 63, 65]. Furthermore, depletion of lens GSH with age has been reported in rats [82] as well as in humans [113, 118]. A decrease in GSH is attributed to various factors, including inactivation of GR by disulfide bond formation and protein unfolding, decreased de novo synthesis, its use in detoxification, increased breakdown, or the failure to regenerate GSH from the oxidized form of GSSG [119, 120].
Depletion of GSH leads to oxidation of protein sulfhydryl groups, resulting in the formation of intra- and inter-molecular cross-links. An increase in disulfides has been reported in aging lenses due to a diminished GSH pool [121]. Mixed protein–thiol and protein–protein disulfide bonds have also been reported in lens proteins [81, 82, 122]. Damage to lens epithelial and fiber cells in early postnatal mice, due to GSH depletion, has been observed [123, 124], but was prevented by pretreatment with GSH prodrugs like glutathione ester [124]. Lou et al. reported a negative correlation between the level of GSH and nuclear opacity [89]. Formation of disulfide bonds, due to depletion of GSH, is believed to be an initiating event in the pathogenesis of cataract formation following oxidative stress [82].
The lens nucleus is highly susceptible to oxidative damage with age. Increased proportions of oxidized GSH in the nucleus are observed in older lenses as well as in nuclear cataracts [84, 86]. However, GSH levels in the outer cortex of the lens remain high, indicating local oxidation and reduced capacity of repair in the nucleus with age. With age, the lens enlarges and its cytoplasm stiffens, resulting in a lower rate of diffusion along with an increase in the distance that reduced GSH must diffuse in order to reach the nucleus [125].
Involvement of oxidative stress in cortical cataracts is not clear. Disruption of fiber cell membranes, followed by disintegration of its cytoplasmic content, occurs in cortical cataracts. It is not clear whether oxidative damage causes disruption of the fiber cells or if it occurs as a result of the loss of fiber cell membrane integrity. Disruption of the plasma membrane would allow GSH and other antioxidants to diffuse out of the fiber cells, resulting in the oxidation of the remaining cytoplasmic content.
Ascorbic Acid (Vitamin C) An aqueous-phase antioxidant, ascorbate, plays a major role in protecting against oxidative stress, especially in a lens where its concentration (1 mM) [126] is 30–35 times that of the plasma [127]. In addition to its antioxidant effects, its high concentration in the aqueous humor in diurnal animals protects the lens against the cataractogenic effect of ultraviolet (UV) radiation in sunlight by absorbing the radiation [128]. Ascorbate is a water-soluble reductant that not only absorbs UV light, but reacts with hydrogen peroxide, oxygen, and free radicals of oxygen [127]. A high level of ascorbic acid decreases the amount of oxygen in the vitreous by reacting with it and forming dehydroascorbate, which spontaneously hydrolyzes, thereby preventing the regeneration of ascorbate by cellular metabolism. Lower ascorbate levels and decreased oxygen consumption have been reported in patients with advanced vitreous liquefaction or previous vitrectomy due to the increased mixing of the vitreous, which exposes more of the ascorbate to oxygen. Vitamin C supplements have been reported to delay or prevent cataracts in several studies [129–132].
Vitamin E Vitamin E, one of the major chain-breaking, lipid-soluble antioxidants of cellular membranes, has four common forms: α-tocopherol, β-tocopherol, γ-tocopherol, and δ-tocopherol. Among these, α-tocopherol is the most effective scavenger of free radicals. The concentration of Vitamin E in the lens, unlike ascorbate, is very similar to that in plasma [133]. However, due to its lipid soluble nature, it is primarily concentrated in the lens fiber membrane, increasing its concentration by several orders of magnitude [134]. Vitamin E is a component of the total ROO• radical trapping antioxidant system [135]. It protects against cataracts by (a) reacting directly with ROO• and O2 •− radicals and singlet oxygen, preventing photoperoxidation of lens lipids, and stabilizing lens cell membranes [136, 137] and (b) enhancing GSH recycling, thereby maintaining reduced GSH levels in the lens and aqueous humor.
Carotenoids Like Vitamin E, carotenoids are natural lipid-soluble antioxidants. Lutein, zeaxanthin, and riboflavin, the major carotenoids in the lens that are related to lens health, act as antioxidants by quenching singlet oxygen and triplet sensitizers, interacting with free radicals, and preventing lipid peroxidation. Low oxygen tension is reported to enhance the antioxidant activity of carotenoid’s, which act synergistically with other antioxidants, like Vitamin C, to protect against oxidative damage to the lens.
18.4.2 Enzymatic Defenses
Several key antioxidant enzymes help maintain the redox status in the lens. These include superoxide dismutase (SOD), catalase, glutathione peroxidase (GPx), and glutathione reductase (GR). SOD represents the only enzyme family with activity against O2 •− radicals. Eukaryotes possess two SOD isoforms: 80-kDa tetrameric Mn-SOD in mitochondria and the 32–34 kDa dimeric Cu/Zn-SOD in the cytosol [138]. Mitochondrial SOD is extremely important in combating ROS, as O2 •− is formed in relatively high concentrations in the mitochondria due to the leakage of electrons from the respiratory chain. SOD converts O2 •− radicals into a less reactive species, H2O2, which is detoxified by two enzymes: catalase and GPx. Catalases are heme-containing enzymes which decompose H2O2 to water and oxygen and specifically use H2O2 as a substrate. On the other hand, GPx (a selenoenzyme) can decompose H2O2 as well as other organic peroxides and requires GSH. The proposed catalytic mechanism [139] involves oxidation of the active site selenolate (Se−) to selenenic acid (SeOH), which is transformed to a selenenylsulfide adduct with glutathione (Se-SG) upon addition of one molecule of GSH. Active selenolate is regenerated by the addition of a second molecule of GSH, resulting in the formation of GSSG. In addition, H2O2 can be degraded nonenzymatically by reduced GSH. The resulting GSSG is converted back to its reduced form by GR using NADPH as a cofactor [83]. In addition to the enzymes that help maintain the normal redox status of the lens, glucose-6-phosphate dehydrogenase (G6PD) is a major enzyme involved in protecting against ROS. G6PD catalyzes the first reaction of the pentose phosphate pathway, which is the conversion of glucose-6-phosphate to 6-phosphoglucono-δ lactone by reducing NADP+ to NADPH. A second molecule of NADPH is generated in the next step of the pathway, catalyzed by another enzyme, 6-phosphogluconate dehydrogenase (6PGD). By these reactions, the cellular level of NADPH that is required for biosynthetic processes and protection against oxidative damage in the lens, are sufficiently maintained. Under conditions of oxidative stress, the pentose phosphate pathway increases to meet the higher demands of NADPH needed by the GSH protective system. However, a decreased efficiency of the pentose phosphate pathway can make the lens more vulnerable to oxidative injury.
Redundancy in the antioxidant defense enzymes provides additional protection against harmful peroxides and, therefore, animals lacking either catalase or GPx-1 appear normal [140, 141]. Interestingly, sensitivity to oxidative stress varies in these knockout animal models, with lenses from GPx-1 null mice being a little more sensitive to oxidative stress than the catalase knockout mice. Furthermore, lenses from SOD-1 (CuZn-SOD)-null mice appear normal, but they are more prone to develop photochemical cataracts [142].
18.4.3 Repair Enzymes
Repair enzymes play a key role in minimizing oxidative stress, along with antioxidant enzymes, by constantly dethiolating the protein–thiol mixed disulfides (protein thiolation) or protein–protein disulfides that result from oxidative insults. This helps restore the functions and activities of lens proteins. Oxidative stress can cause several protein modifications, including oxidation of methionines, oxidation of cysteines, and cross-linking of proteins through amino acids other than cysteine, which results in high molecular weight insoluble aggregates that are characteristic of cataracts [84, 143, 144]. The following three enzymes play a major role in the repair of proteins with oxidative damage:
GSH–Dependent Thioltransferase (TTase) or Glutaredoxin (GRx) This is a member of the thiol-disulfide oxidoreductase enzyme family with two isozymes: cytosolic TTase-1 (GRx1) and mitochondrial TTase-2 (Grx2) [145, 146]. It catalyzes the dethiolation of protein–thiol mixed disulfides [147] by using GSH as a cofactor. It is essential for maintaining the thiol-disulfide homeostasis in the lens by repairing the thiolated proteins [148], regulating GSH-dependent protein activity [149], and by preventing lens proteins from cross-linking.
Since thiolation is an initial way of protecting proteins from irreversible damage, a delicate balance between thiolation and dethiolation of proteins is crucial; TTase-1 is a key enzyme involved in the regulation of the ratio of intracellular SH/-S-S. TTase is a major antioxidant defense enzyme that is distributed in most ocular tissues [150]; however, it is two to threefold times higher in the epithelial layer. The iris has been shown to have the highest activity, followed by conjunctiva, corneal epithelial cells, and corneal endothelial cells [89]. Moderate activity has been demonstrated in the ciliary body, lens epithelial cells, and the retina, but none has been detected in the vitreous body. A striking feature of this enzyme is that it is resistant to oxidative stress under conditions where other thiol sensitive defense enzymes fail [151]. A higher concentration of this enzyme in the anterior segment of the eye (which is more vulnerable to oxidative stress) indicates the crucial protective role of TTase against oxidative stress-induced damage. In addition to dethiolation, these enzymes also act as dehydroascorbate reductase and reduce dehydroascorbate [152] in the presence of GSH. These enzymes prevent cataract formation by reducing dehydroascorbate (DHA), which is known to be toxic to the lens and can induce cataract formation [153]. Overexpression of thioltransferase in human lens epithelial cells (HLE B3) protected against oxidative stress induced by low levels of H2O2 [154]. In addition, Grx2 prevented disruption of the mitochondrial transmembrane potential during oxidative stress in lens epithelial cells [155].
NADPH–dependent thioredoxin/thioredoxin reductase system (Trx/TR system) This system consists of two antioxidant oxidoreductases, namely: thioredoxin (Trx) and thioredoxin reducatse (TrxR). Trxs are small (10–12 kDa), ubiquitous proteins that catalyze the dissociation of disulfide bridges between proteins [156] and the reduction of protein thiols that have been oxidized to sulfenic acid [157]. The redox activity of their conserved active-site motif (Cys-Gly-Pro-Cys-) is crucial for their function as an oxidoreductase [158]. Oxidized thioredoxin is, in turn, reduced by selenoenzyme TrxR that uses NADPH as a cofactor [159]. TrxR isozymes are homodimers with one FAD per subunit that reduces the active site disulfide in an oxidized Trx [158, 160].
Trxs play numerous roles, including supplying reducing equivalents to enzymes like ribonucleotide reductase, methionine sulfoxide reductase, etc., selectively activating transcription factors, altering gene transcription, and functioning as cell growth factors, and also as inhibitors of apoptosis [161–164].
Oxidative stress induces the expression of Trx [165, 166], which is believed to protect against oxidative stress by (a) modulating signal transduction properties of ROS [167, 168], (b) scavenging radicals [169], (c) reducing intracellular disulfides, (d) selectively modulating the DNA-binding of transcription factors like AP-1 [170, 171] and NF-kB [172], (e) preventing apoptosis by binding to apoptosis signal-regulating kinase 1 (ASK-1) [173], (f) donating electrons to periredoxins, which are involved in the reduction of peroxides [174, 175], (g) reactivating partially oxidized and inactivated recombinant TTase [148], and (h) selectively inducing several antioxidant gene expressions [176].
TRx, a vital repair protein in the lens, is present in the epithelial, cortical, and nuclear regions of human lenses. However, the epithelial layer possesses three times more TRx 1 protein [159] than the other two regions since this layer is the initial target of oxidative insult. TrxR is present in the epithelial, as well as in the fiber cells of human lenses [177]. Upregulation of TRx 1 under oxidative stress was reported in an Emory mouse lens exposed to photooxidation [178] and in HLE B3 cells exposed to H2O2 [159]. This was believed to play a significant role in protecting the lens against oxidative insults [159]. Decline in the expression of the human lens thioredoxin gene and its protein in the human lens, with advanced age, indicates an association between altered Trx1 and senile cataractogenesis [177].
Methionine sulfoxide reductases (Msrs) Msrs are a family of thioredoxin-dependent oxidoreductases that catalyze reduction of oxidized methionine to methionine [179]. Two classes of Msrs, MsrA and MsrB, have been reported, which repair S- and R-epimers of methionine sulfoxide (MSO), respectively [180].
Oxidation of methionine to methionine sulfoxides has been reported in cataractous lenses [62, 64] and has been implicated in the etiopathogenesis of cataracts. Repair of oxidized methionine has been shown to protect against oxidative stress since oxidation of methionine may lead to significant changes in protein structure and functions [180–182]. Msr activity has been reported in lenses [179, 183] and both classes of Msrs protect lenses against oxidative stress-induced damages [183, 184]. Marchetti et al. provided evidence that MsrA is essential for cell viability and silencing it resulted in the loss of mitochondrial membrane potential and increased ROS levels in human lens cells [185]. Furthermore, Kantorow et al. reported high levels of MsrA transcript and protein throughout the human lens and showed that MsrA was essential for lens cell viability and protected the lens even in the absence of oxidative stress [183]. Overexpression of MsrA in lens epithelial cells was reported to protect against H2O2 mediated oxidative stress, whereas silencing of the MsrA gene using siRNA increased sensitivity to H2O2 mediated oxidative stress in lens cells. In addition, MsrB which contributes about 40 % of Msr activity in the lens played an important role in protecting against tert-butylhydroperoxide (tBHP)-induced oxidative stress [184].
Homeostasis of thiol/disulfide in cells is synergistically regulated by the TTase and TRx systems [158]. There is cross talk between the Trx/TrxR and TTase systems, as evidenced from the finding that Trx can induce TTase1 and TTase2 mRNA expressions. TTase dethiolates protein–thiol mixed disulfides, whereas TRx repairs enzymes and proteins that are oxidized to sulfenic or sulfunic acids. These enzymes act to restore cellular functions and energy production and, thereby, protect cells under oxidative stress. However, their activity is reported to decrease with age, which is one of the risk factors for cataracts.
Thus, a lens has several layers of primary protective mechanisms that help protect it against oxidative stress. However, when oxidative stress becomes overwhelming, a diminished pool of reduced GSH may cause a decrease in the ratio of the GSH/ GSSG, together with an impaired repair system. A decrease in total protein thiols, together with increases in protein–thiol mixed disulfides (PSSG and PSSC) and protein–protein disulfides result in the formation of high molecular weight protein aggregates, which are water insoluble and eventually cause light scattering and cataract formation.
18.5 Treatment Options
18.5.1 Surgery
The most common indication for cataract surgery is the patient’s desire for improved visual function. Surgery is not based on a specific level of visual acuity. The patient and the physician determine whether the reduced visual function interferes with the adult’s daily living (ADL) parameters [186].
Preoperative evaluation includes assessment of the general health of the patient, pertinent ocular history, social history, and postoperative care. The examination starts with an external exam and is followed by slit lamp examination of the conjunctiva, cornea, anterior chamber, iris, and lens. Fundus examination must be performed to assess any posterior segment pathology. After a decision is made that the cataract surgery is indicated to improve visual function, preoperative measurements with biometry, corneal topography should be done to determine the intraocular lens implant power. Topical anesthesia mostly used during cataract surgery but, depending on patient factors, local or general anesthesia can be preferred.
Extracapsular cataract extraction by nucleus expression involves removal of the lens nucleus and cortex through an opening in the anterior capsule, leaving the capsular bag in place. Phaco-emulsification is an extracapsular technique that uses an ultrasonically driven tip to emulsify the lens nucleus and aspirate the lens.
An incision is made in the sclera or clear cornea with a blade and special viscoelastic materials are used to maintain the intraocular space during the surgery. A circular opening is created in the anterior capsule and a phaco tip is inserted through the scleral or corneal incision toward the lens. There is continuous irrigation through the phaco tip so that ocular volume is maintained. The lens cortex is emulsified by the phaco handpiece, which oscillates at a frequency of 27,000–60,000 Hz. The melt is then aspirated through the handpiece. A femtosecond laser (1,053 nm wavelength) has been developed for creating corneal incisions, performing anterior capsulotomy, and fragmenting the nucleus; however, it is currently unable to completely emulsify the nuclear material. After the cortical and epinuclear materials are removed with a different handpiece, an intraocular lens implant is inserted within the capsular bag.
Today’s intraocular lenses (IOLS) have evolved considerably since the initial implant in 1949. Harold Ridley, an English ophthalmologist, observed that the polymethylmethacrylate (PMMA) fragments from an airplane cockpit windshield embedded in the eyes of pilots were tolerated in the anterior segment of the eyes [186]. This led to the idea that, when cataractous lens is removed, refractive power of the eye can be restored using an intraocular lens.
IOLS have undergone significant changes in geometry (loops of different length, stiffness, vault and angle configuration; optics of different diameter, holes, style, and shapes) [186]. Then, with the advent of small incision cataract surgery, came the foldable IOLS. The majority of current IOLS is foldable and is either silicone or acrylic.
Monofocal IOLS have the drawback of a fixed focal point. Newer multifocal IOLS now offer refractive correction for near, intermediate, and distance vision at the expense of decreased contrast sensitivity, glare, and halos. Most IOLs have ultraviolet absorbing chromophores to protect the retina from radiation, while some IOLS have blue-blocker to prevent long-term damage to the retina caused by high frequency blue light.
The refractive power of IOLs should be specifically measured for each eye. IOL calculation is prone to some degree of error, but Light Adjustable Lens (Calhoun Vision) has currently been developed to circumvent this problem. The optic of the three piece foldable silicone lens is composed of an unpolymerized silicone matrix polymer, a polymerized macromer, a photo-initiator, and a UV-absorber. A digital light delivery device induces polymerization of the unpolymerized silicone. Irradiating the centre of the lens decreases the central curvature, with a resulting hyperopization of the IOL’s refraction, while irradiating the periphery of the IOL increases its central curvature, with a resulting myopization. After a few weeks, a final lock-in of the IOL power is achieved [187].
Cataract Surgery Outcomes Modern cataract surgery has a very satisfying success rates as indicated by one large study where 95 % of adults were satisfied with the results of their surgery. Some tools assess how visual function is affected, including the VF-14 questionnaire and the Activities of Daily Vision Scale (ADVS), which is a measure of vision specific functional status [186]. Studies have shown significant improvement in the quality of life parameters such as driving, mental health, life satisfaction, and community and home activities [186].
Complications of Cataract Surgery Complication rates of modern cataract surgery are low, less than 5 %. Problems may occur during the surgery or in the immediate or late postoperative period. Intraoperative complications are wound related like Descemet’s membrane detachment, thermal burns of the wound, and tears or breaks in the capsulotomy which may lead to extension of a tear toward the posterior capsule with loss of lens material to the posterior segment. Others are iris prolapse, hemorrhage in the retrobulbar space, anterior segment or suprachoroidal hemorrhage, and retained lens material. Some of these can be salvaged without loss of vision, while others like suprachoroidal hemorrhage may have grim consequences. Postoperative complications are wound dehiscence, wound leakage, corneal edema and bullous keratopathy, hemorrhage, uveitis, increased intraocular pressure, induced astigmatism, IOL decentration or dislocation, wrong IOL power, endophthalmitis, cystoid macular edema, macular infarction, retinal detachment, posterior capsule opacification, and epithelial downgrowth [188]. Although, cataract surgery is generally very successful, it should be kept in mind that it is not a risk-free procedure.
18.5.2 Alternative Treatment Options: GSH Prodrugs
Cataracts are routinely treated by surgical replacement of lens, a safe and effective approach. Although this surgery is currently the accepted treatment, it requires a significant and growing share of medical resources, especially through Medicare. Over three million procedures are performed in the United States each year at an estimated total cost of about $9 billion. In the face of the rapid increase in the prevalence of cataracts expected over the coming decades, an effective, nonsurgical treatment to prevent and treat cataracts would represent a cost-effective therapeutic alternative. Investigators at the Tufts University School of Nutrition estimate that delaying the onset of cataracts by 10 years would reduce the number of cataract extractions by half. Since oxidative stress is implicated in the pathogenesis of a cataract, a logical approach to drug development would be the prevention of oxidative damage to the lens. The lens, because of its anatomical location is subjected to significant photooxidative stress, requiring elevated levels of cellular antioxidants to neutralize photochemically generated ROS. In the lens, GSH is the primary cellular antioxidant and plays a critical role in protecting the lens from the toxic effects of ROS. It is found in lens epithelial cells at levels almost twice that of other cells in the human body. A young lens has a high concentration of enzymes, such as ascorbate and GSH, but the ability of the cell to synthesize GSH declines with age. This results in decreased GSH levels that leave the lens more vulnerable to oxidative damage and, eventually, cataract formation. Under conditions of chronic imbalance between antioxidants and ROS, oxidative damage results in structural perturbation of the crystallin proteins that comprise 90 % of the lens tissue and are responsible for maintaining its transparency. Since crystallins are long-lived stable proteins with minimal turnover, accumulation of oxidative damage leads to protein cross-linking, aggregation, insolubility, and fragmentation, resulting in the formation of cataracts. The important role of ROS and resulting oxidative damage in the development of various types of cataracts has been established by a number of studies.
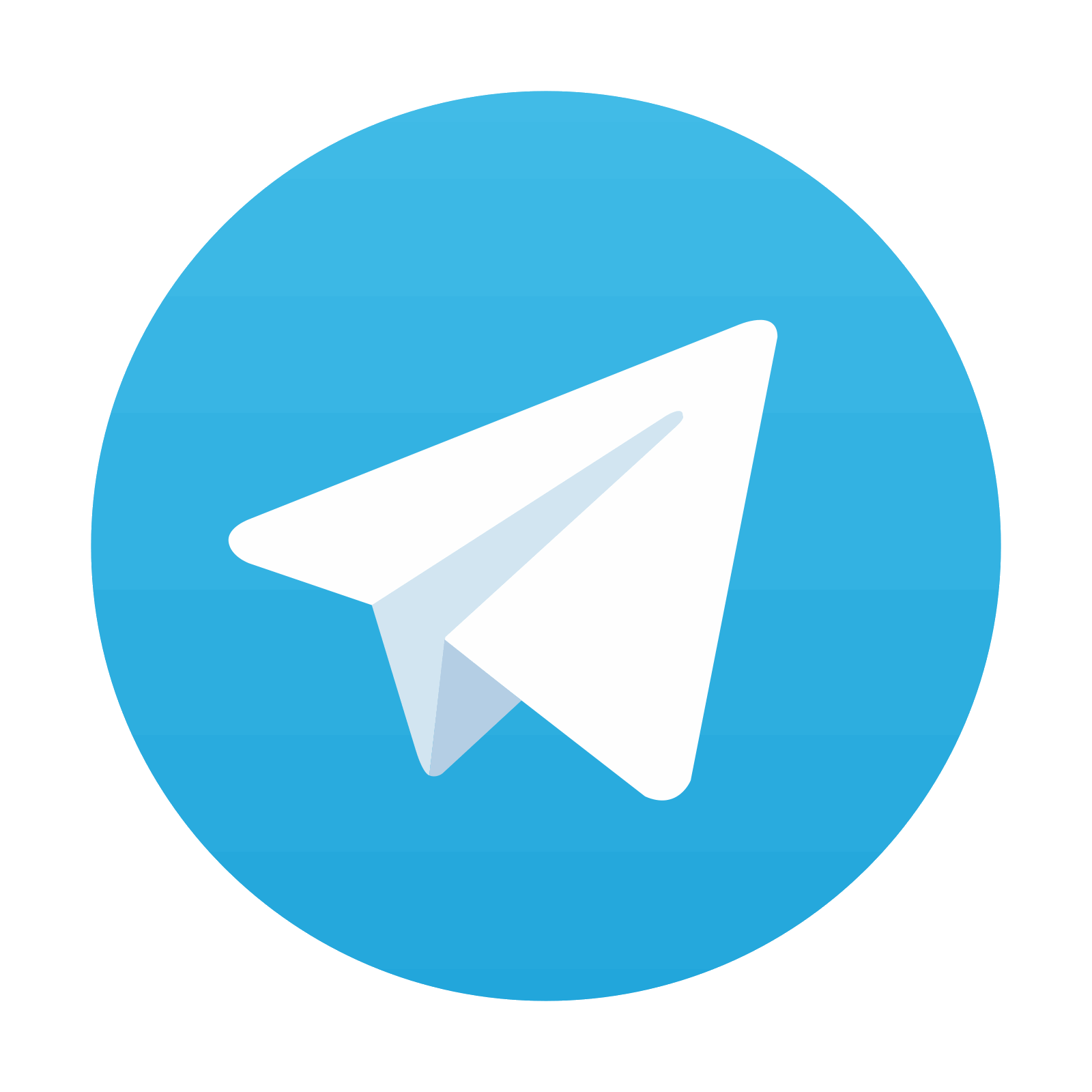
Stay updated, free articles. Join our Telegram channel
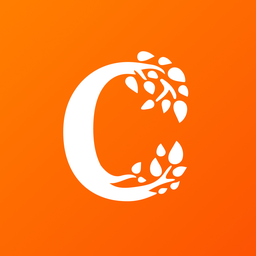
Full access? Get Clinical Tree
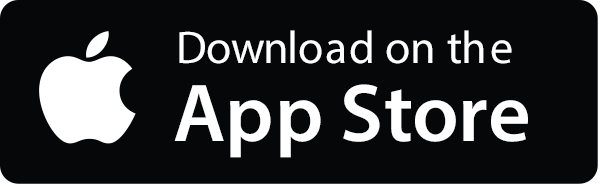
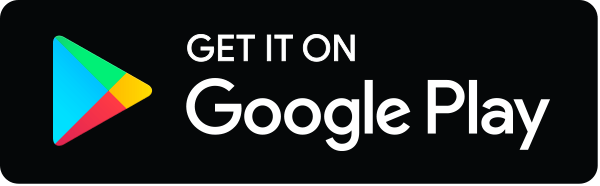