Fig. 65.1
Anatomical structure of the developing embryo. (a) displays a quail embryo imaged at 12× magnification. (b) shows a B-scan image of the quail embryo heart imaged by OCT. The green dotted line approximates the location of the B-scan. OCT allows visualization of all layers in the heart tube (myocardium, cardiac jelly, and endocardium) in both the inflow and outflow regions. (c, d) Doppler OCT data are overlaid on a structural B-scan of the outflow tract during diastole and systole. The red dotted line in B gives the approximate location. The color map is shown to the right of the image. Myo myocardium, CJ cardiac jelly, BL blood, Endo endocardium
Early demonstrations of imaging the developing heart using OCT demonstrated its ability to visualize cardiac dynamics in one and two spatial dimensions versus time [20–23]. 1-D and 2-D imaging over time opens up the possibility of many exciting experiments. However, biomechanical forces acting upon the heart are often spatially heterogeneous and varying in time. For example, not only does shear stress on the endocardium vary greatly along the length of the heart tube, but certain regions experience oscillatory shear due to regurgitant flow [10]. In order to fully assess complex patterns of biophysical forces, it is necessary to image the beating heart with high spatiotemporal resolution in four dimensions (x, y, z, and time). This is what we refer to as 4-D imaging. Cardiac function and the resultant forces are constantly evolving throughout development. Biomechanical cues are vital for proper growth and maturation. Shear stress on and stretch of the endocardium, strain in the myocardium, and electrical signals have all been implicated as necessary for normal development [6–15]. Also, small deviations in biomechanics can signify that the embryo is developing a CHD. Deciphering the complex spatial and temporal patterns of biomechanical forces acting upon the heart is necessary to fully understand the role of function in the developing embryo.
Numerous solutions over the past several years have demonstrated 4-D OCT imaging of the developing cardiovascular system. This chapter will focus on these solutions and explain their context in the evolution of 4-D OCT imaging. The first sections describe the relevant techniques (prospective gating, direct 4-D imaging, retrospective gating), while later sections focus on 4-D Doppler imaging and measurements of force implementing 4-D OCT Doppler. Finally, the techniques are summarized, and some possible future directions are discussed.
65.2 Prospective Gating
Cardiac-gated imaging enables the capture of 4-D image data sets by mitigating motion artifacts when the temporal resolution of the system is inadequate for direct 4-D imaging (real-time volumetric imaging). Because cardiac motion is highly repetitive, one can build up a 4-D image set of the beating heart over multiple beats. For prospective gating, a physiological signal is used to trigger data acquisition at specific phases of the cardiac cycle. After several heartbeats, one collects data of the beating volumetric heart by acquiring more data at each cardiac phase with each subsequent heartbeat. Prospective gating has been implemented in several medical imaging modalities found in the clinic including MRI and CT [24, 25].
In 2006 Jenkins et al. used a prospective gating technique to capture 4-D OCT images of beating avian and murine hearts [26]. Because of the difficulties of obtaining a steady physiological trigger signal, the heart was excised and paced as a first step to prove the principle. Specifically, hearts were excised in a Tyrode solution and placed between two platinum plates. A 50 ms electrical pulse both paced the heart and triggered data acquisition. The Tyrode bath was kept at room temperature which lowered the heart rate and enabled pacing at 1 Hz. A time domain OCT system with a bandwidth centered around 1,300 nm acquired data at 4,000 A-scans/s with an axial resolution of 14 μm and a lateral resolution of 10 μm. Sixteen cardiac volumes were captured per heartbeat with imaging of one heart lasting less than 5 min. Chicken embryos were imaged at stage 13, 15, and 20, corresponding to 2–3 days of development, while mouse embryos were imaged on day 13.5. Images were averaged and filtered to reduce noise before correcting the aspect ratio and creating surface renderings of the 4-D structures. Measurements of cardiac volume, ejection fraction, and wall thickness were demonstrated, although some of the measured values probably differ from in vivo measurements made under physiological conditions. This work demonstrated the feasibility of 4-D OCT imaging of a paced, excised heart, but not of the beating heart in an intact, living embryo.
Obtaining ECG signals from patients in the clinic is a simple procedure and can easily be utilized to gate image acquisition. Unfortunately, obtaining a proper ECG signal from an early stage embryo is both difficult and invasive. To overcome this challenge and enable in vivo 4-D imaging, Jenkins et al. designed a prospective gating technique by triggering data acquisition with a laser Doppler velocimetry (LDV) signal [27]. Briefly, a commercial LDV needle probe (Moor Instruments Incorporated, Wilmington, Delaware) was positioned adjacent to a vitelline vessel of a stage 14 quail embryo cultured on the yolk in a Petri dish on a temperature-controlled heating pad (37 °C). The choice of vitelline vessel was not important as long as a strong signal was obtained. The signal was conditioned and then directed to a commercial R-wave detector (AccuSync Medical Research Corporation), which produced two triggers: one at the peak of the LDV signal and one at the bottom of the downward slope. Finally, a field programmable gate array (FPGA) received inputs from the R-wave detector and a line-sync from the OCT delay line to produce a frame sync and to control the scanning. The OCT system was identical to the one used in the previous study. Eight heart volumes were collected. Figure 65.2 displays 2-D cross-sections and 3-D volumes (digitally reconstructed radiographs) of a stage 14 quail embryo heart in vivo during systole and diastole. Excellent initial results were demonstrated with prospective OCT gating using LDV, but the requirement of an external signal is a disadvantage relative to direct 4-D imaging and signal-free retrospective gating (which have become feasible using current, high-speed OCT systems) due to decreased set up time. However, prospective gating does require less computation time than retrospective gating which can be advantageous for certain applications.
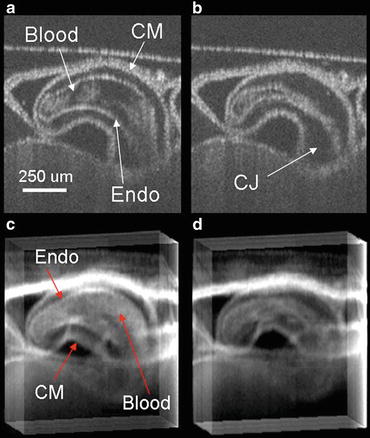
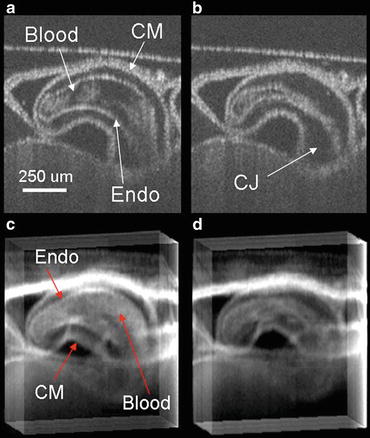
Fig. 65.2
Prospective gating employing a laser Doppler velocimetry (LDV) probe. (a) OCT B-scan acquired during diastole cut coronal to the body of the embryo. (b) OCT B-scan acquired during systole cut coronal to the body of the embryo. (c) Digitally reconstructed radiograph image acquired during diastole. (d) Digitally reconstructed radiograph acquired during systole. Digitally reconstructed radiographs project signal intensity along the sagittal orientation. CM is the compact myocardium, endo is the endocardium, and CJ is the cardiac jelly
65.3 Direct 4-D Imaging
Direct 4-D imaging is defined as real-time volumetric imaging without the aid of gating techniques. Until recently, OCT systems were not capable of 4-D imaging of embryo hearts without gating due to insufficient imaging speed, but as speeds have dramatically increased, 4-D imaging has become feasible with limited spatiotemporal resolution. This step forward is attributed to the paradigm shift to frequency-domain OCT systems and the development of high-speed swept laser sources [28–33]. Details of these developments are presented in other chapters of this book.
Jenkins et al. demonstrated direct 4-D imaging of embryonic quail hearts using a Fourier domain mode-locked (FDML) laser [34]. The high-speed swept source allowed imaging speeds of 100,000 A-scans/s. The axial resolution in tissue was 7 μm, while the lateral resolution was 15 μm. 4-D imaging of stage 14 quail embryos was collected at ten volumes/s (70 × 150 A-lines/volume). Embryos were cultured on a yolk in a Petri dish and imaged on a temperature-controlled plate (37 °C). Because the embryos were not imaged in an incubator, the heart rates were slightly depressed (∼2 Hz), resulting in 4–6 volumes per heartbeat. Several visualization techniques were also demonstrated, including digital reconstructed radiographs, Sobel filtering, and multiplanar reformatting. Figure 65.3 displays the top view of a stage 14 quail embryo heart where a 3-D Sobel mask was applied to the data to bring out the edges of the structures. In 2008, Gargesha et al., using a similar system, demonstrated that volume visualizations applying a 2-D opacity transfer function and image denoising could improve 3-D visualizations and automated segmentation of 4-D data sets [35]. Considering that the maximum wall velocity can reach almost 2 mm/s, a volume must be imaged in 5 ms in order to reduce motion artifact below 10 μm [34]. The authors noted that in order to accurately capture the 3-D motion of the heart tube without gating, faster imaging systems were needed.
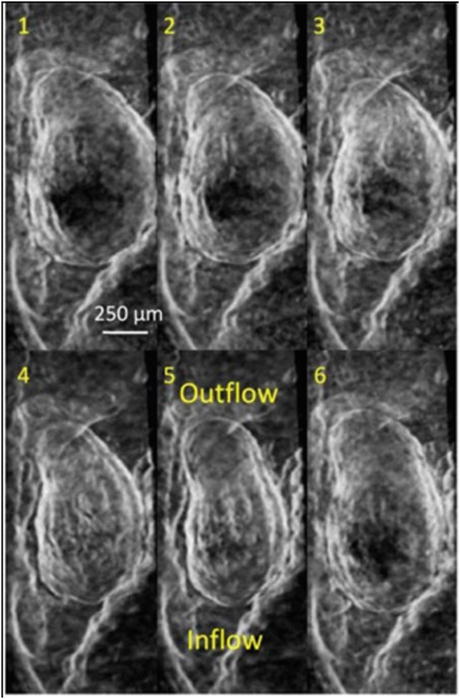
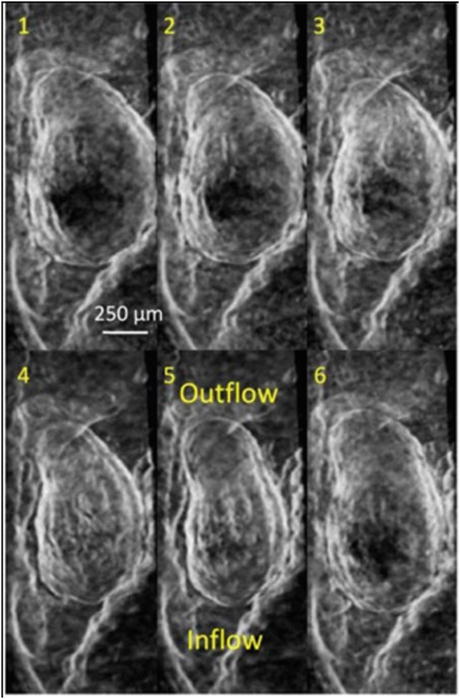
Fig. 65.3
Top view (ventral) of a beating stage 14 quail heart (six phases in the cardiac cycle). Data are visualized as an edge-enhanced volume rendering (Data was collected through 4-D direct imaging)
Yelin et al. demonstrated 4-D OCT imaging of stage 49 Xenopus laevis hearts using a wavelength-swept laser with a polygon scanner-based spectral filter [36]. 54,000 A-scans/s were captured allowing for 20 volumes/s with each volume consisting of 60 × 45 × 256 pixels. The axial resolution was 10 μm in tissue. Embryos were covered by an anesthesia solution and positioned ventral side up on an agar plate. Displacement maps were constructed to show myocardial motion at different phases in the cardiac cycle. Again, although 4-D imaging was demonstrated, meaningful measurements were not possible because of the limited speed of the system.
Direct 4-D OCT imaging is attractive for several reasons which include decreased procedure time, reduced post processing, no increased noise due to abnormal beats, and the ability to capture nonperiodic events like arrhythmias. Over the last several years, FDML lasers have increased OCT imaging speed dramatically including a demonstration of 20 MHz A-line rates [37]. This extraordinary increase can enable direct 4-D imaging with sufficient spatiotemporal sampling (e.g., volumes 2 mm × 1.25 mm transversely in 5 ms) and obviate the need for gating.
Unfortunately, an OCT system this fast is very complex and expensive. Data acquisition and handling are extremely challenging, and adequate commercial DAQ products are not presently available. Real-time data visualization at these rates is also a challenge. Several demonstrations have been reported of real-time 4-D OCT display of tissue structures utilizing graphics processing units (GPU) [38–40]. Although these systems need to improve data throughput to visualize the developing embryo heart in a meaningful way, it is likely that new developments in OCT technology and GPUs will enable this in the future.
4-D Doppler OCT imaging will remain difficult without gating. Doppler OCT requires denser spatial sampling than is needed for structural OCT, slowing imaging speed. Also, because scanning speed dictates the detectable velocity range, capturing 4-D Doppler datasets would be require innovative scanning patterns.
65.4 Retrospective Gating
65.4.1 Signal-Based Retrospective Gating
A signal-based retrospective gating scheme records a physiological signal simultaneously with the image data. Unlike prospective gating where the physiological signal gates data acquisition, here the signal is utilized in a post processing algorithm to parse the data into the appropriate phases of the cardiac cycle creating a 4-D data set. Yazdanfar et al. first demonstrated retrospective gating of a Xenopus laevis heart on an OCT system with a scanning rate of 8 A-scans/s [41]. By utilizing the structural OCT signal to determine the number A-scans per heartbeat, the authors obtained five Doppler and structural frames per heartbeat. Building on this first demonstration, Mariampillai et al. implemented a signal-based retrospective gating technique to capture both 4-D structure and Doppler images of stage 51 Xenopus laevis hearts [42]. The system comprised two OCT systems; one an adapted commercial swept source OCT system (Thorlabs, SL1325-P16) for imaging and the second a time domain OCT system to capture a Doppler optical cardiogram used as the gating signal. The swept source system had an A-line rate of 16 kHz and was capable of real-time imaging at 25 fps (structural) and 12 fps (Doppler). Briefly, stage 51 Xenopus laevis embryos were anesthetized with lidocaine and placed in a shallow V-groove with solution. The time domain OCT system with a rapid scanning optical delay (RSOD) line had an A-line rate of 12.95 kHz. The system recorded from one of the two vessels exiting the heart and operated in M-mode (no scanning). The M-mode Doppler signal was processed, and the mean and standard deviation of the heart rate were calculated from the optical cardiogram, and cardiac cycles with variations below 1.75 standard deviations were kept. Next, the cardiac cycle was separated into equally spaced temporal bins. B-scans acquired from the swept source OCT system were placed into the correct bin by time stamp and reassembled. Finally, 4-D surface reconstructions of both the structure and Doppler images were presented. Signal-based retrospective gating has some promise, but like prospective gating it requires a more complex technical setup and additional setup time between embryos and has lost favor among research groups investigating embryonic cardiac dynamics in favor of signal-free gating.
65.4.2 Image-Based Retrospective Gating
Image-based retrospective gating (or signal-free, i.e., not requiring an external signal) has become the current standard for 4-D OCT imaging in developmental cardiology studies [43–45]. This technique records images continuously, without gating, over several heart cycles, then rearranges out-of-order data based upon image similarity and obviates the need for extra signals to synchronize data. Although the system complexity is greatly reduced, the gating algorithm requires extra processing time. Gargesha et al. demonstrated 4-D reconstructed imaging of embryonic hearts using an image-based retrospective OCT gating technique by adapting an algorithm originally applied to confocal microscopy images of living zebrafish embryos [44]. The technique consisted of capturing B-scans (500 A-lines) longitudinal to the heart tube at a single location for at least 1.5 heartbeats before translating to a parallel slice 9 μm away. This process was continued until the entire heart was imaged (∼80 slices). The embryos were imaged under physiological conditions by controlling temperature and humidity in an environmental imaging chamber. The scanning orientation was chosen to minimize motion artifacts. The B-scan interval for the system was 4.27 ms. It was previously determined that the maximum error in displacement due to a 5 ms time step was 10 μm [34]. If the first A-scans in the B-scan were captured 5 ms before the last A-scans of the B-scan, there would be a 10 μm displacement error if the velocity of the heart wall was 2 mm/s. Since the maximum wall velocity for stage 14 quail embryo is 2 mm/s, the B-scan interval was fast enough to assume that the motion was frozen during each B-scan.
The gating algorithm consisted of four main steps:
1.
Ascertain the period of the heart cycle.
2.
Determine relative time shifts between adjacent image slice locations.
3.
Establish absolute shifts to align slices with user-selected starting time.
4.
Reorder image data.
These steps are similar for all works discussed in this section unless stated otherwise. Before step 1, the data can be decimated to reduce processing time or alternatively can be synchronized based upon a subset of wavelet coefficients from the image [43, 44]. (1) To calculate period of the heart cycle, a string-length minimization method was applied [46, 47]. Image data at each slice location was wrapped into a single cardiac cycle for each guess of the period. An objective function computed the sum of squared differences between temporally adjacent reordered slices and the sum of squared differences between frame times. The period that minimizes the objective function was chosen, and the data were reassembled into one correctly ordered cardiac cycle. The data were then interpolated to create B-scans evenly spaced in time. (2) The sequence of B-scans at each transverse position has a different starting point in the cardiac cycle so the temporal shift between the transverse positions must be determined. The relative shift in time between adjacent transverse positions was determined by maximizing the cross-correlation coefficient between the images as the relative shift was varied. (3) The absolute shift of each slice location was set corresponding to a user-defined reference slice at the middle of the heart tube. The reference shifts were progressively applied to each consecutive slice moving outward from the middle giving an array of correct starting offsets for each slice position. (4) The determined period and absolute shifts were employed to reorder the data. Figure 65.4 shows an example of data obtained with this image-based retrospectively gated OCT imaging method.
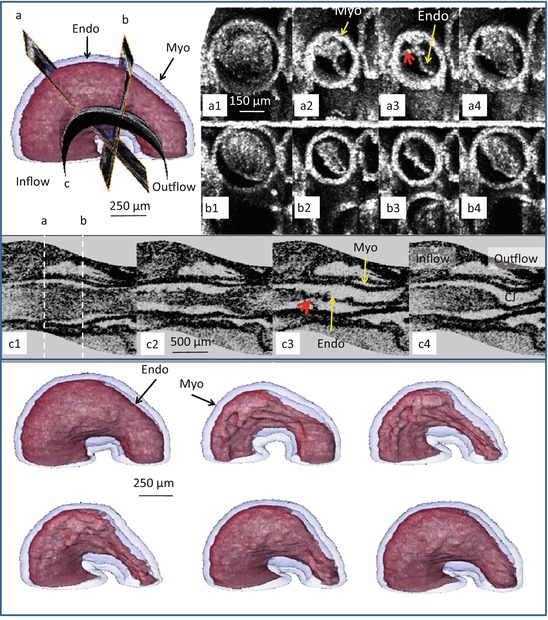
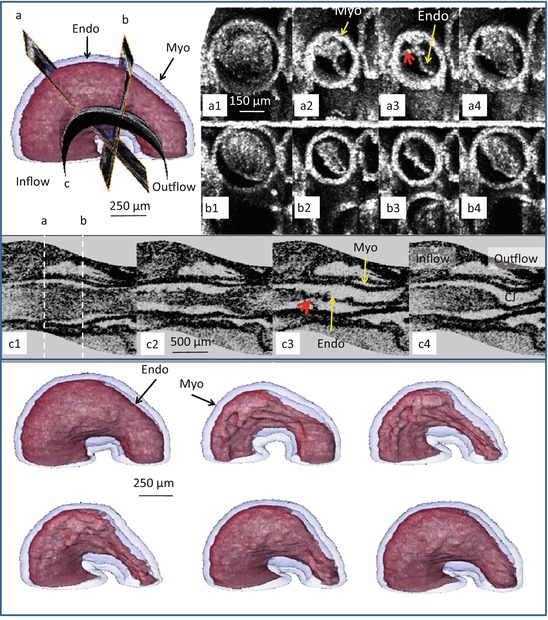
Fig. 65.4
Image-based retrospective gating. Upper left panel displays the location of the three slices (a, b, and c) on the 3-D heart. A1–4 and B1–4 slices at locations (a, b) oriented orthogonal to the heart tube at several different time points during the cardiac cycle. C1–4 illustrates the ability to visualize a curved surface through the center of the length of the heart tube. The locations of slices (a, b) are indicated with the white dashed lines. The bottom panel displays surface renderings as the heart progresses through a cardiac cycle. The endocardium is in red and the myocardium in transparent blue. Myo myocardium, Endo endocardium
Gargesha et al. validated the gating algorithm by comparing the retrospectively gated results to directly recorded volumetric imaging of the same samples [44]. Knowing the time delay between slices in the directly imaged volume, the accuracy of the gating algorithm was determined by finding the slices in the directly imaged volume that correlated best with volumes in the gated image set. The maximum error was 18.7 ms, and the standard deviation of the error was 4.7 ms indicating that the gating algorithm would be capable of producing 200 volumes/s with minimal displacement error (<10 μm). It is likely that this displacement error would increase for OCT systems with slower A-scan rates, and further enhancements in the algorithm would be needed.
Liu et al. demonstrated a similar retrospective gating algorithm to image the outflow tract of stage 18 chicken embryos [45]. A spectral domain OCT system acquired 40 B-scans/s consisting of 250 A-lines. The resolution of the system was 10 μm axial and 16 μm lateral. The eggshell was opened to enable imaging, while temperature and humidity were not controlled and the heart rate was depressed. The Liu algorithm differed from the algorithm described above in several ways. First, instead of maximizing the similarity between adjacent B-scan sequences by varying the relative shift, similarity was maximized between adjacent M-mode images extracted along the same line perpendicular to the B-scans (essentially using one line per image), which increased computational efficiency. To improve accuracy, images were recorded over five cardiac cycles instead of 1.5–2. Furthermore, B-scan slice sequences were transverse to the heart tube. Finally, an extra perpendicular B-scan sequence longitudinal to the outflow tract was utilized to correct the phase lag between adjacent image sequences. Briefly, the phase lag was computed by extracting two M-scans from the sequence of B-scans longitudinal to the outflow tract. By computing the distance between the M-scans and the phase between maximal contractions, the phase lag could be computed. For this measurement, the velocity of the contraction wave was assumed constant in the outflow tract. Reconstructions consisted of 180 volumes per cardiac cycle.
Recently, Happel et al. designed a gating algorithm implementing rotational image acquisition, where each set of B-scan sequences is rotated from one another providing a central A-scan common to all B-scan sequences [48]. The common A-scan was utilized to determine the relative shift between each B-scan sequence. Images were collected with a Thorlabs swept source OCT system (OCS1300SS). Fifty B-scans (>9 images/heartbeat) were acquired in 2.4 s (>5 heartbeats) at every angle (every 2°) at a frame rate of 21 B-scans/s (each B-Scan consisted of 512 A-lines). The axial resolution was 9 μm in tissue, while the lateral resolution was 25 μm. Twenty volumes per cardiac cycle were assembled. Validation was done by acquiring B-scan sequences collected at positions differing from those taken in the 4-D data set and correlating them with the reconstructed data. From Fig. 65.5, one can see that the reconstructed data compares favorably to the validation B-scan sequences.
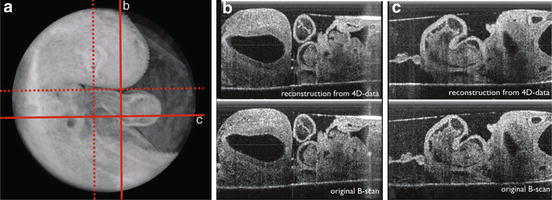
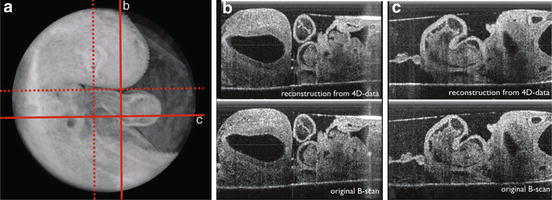
Fig. 65.5
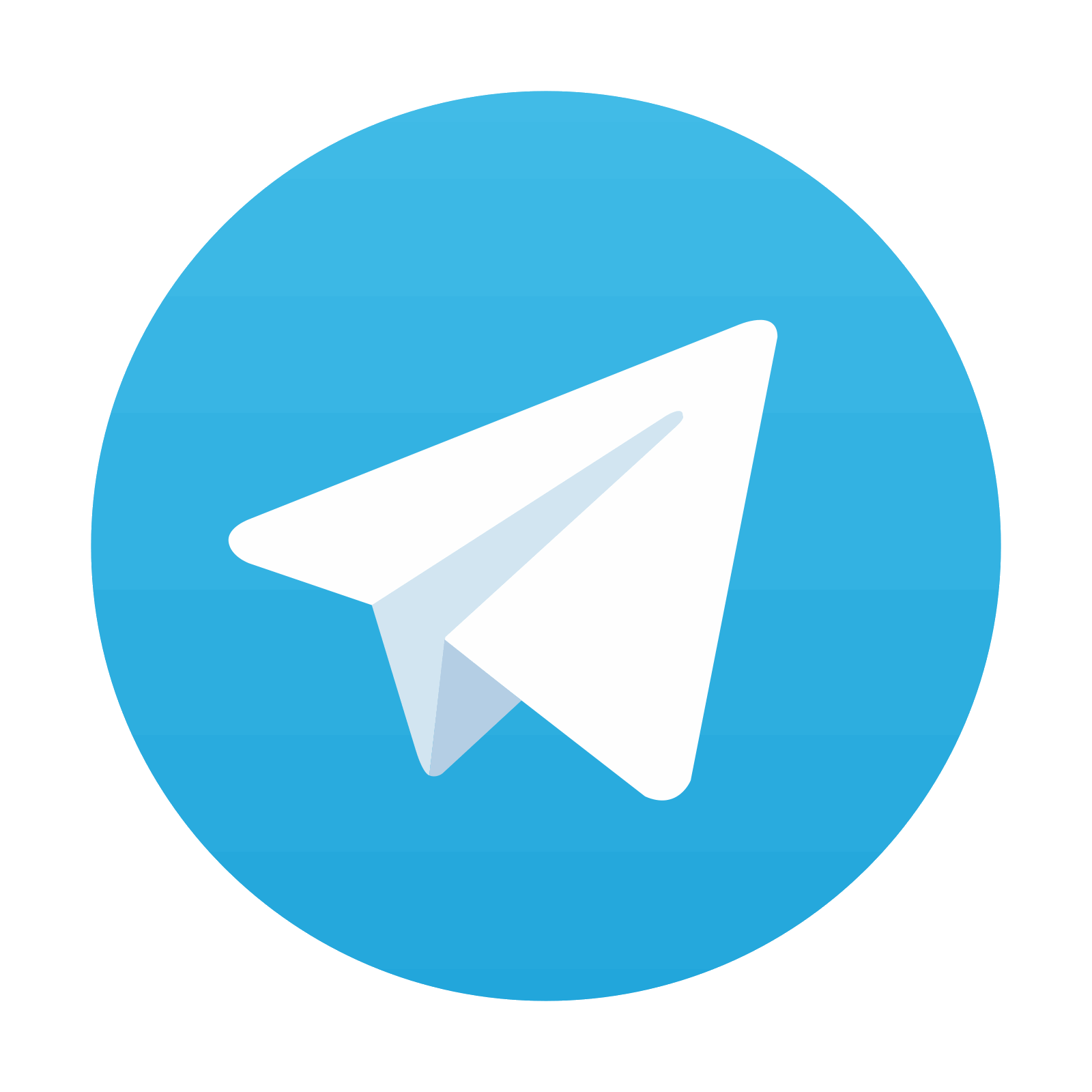
Retrospective gating implementing rotational image acquisition. (a) displays a 4-D image set collected with rotational image acquisition. The red lines indicate the position of b and c. (b) B-scan from the reconstructed 4-D data looks comparable to a standard B-scan at position b taken after the 4-D scan was completed. (c) respective images from position c
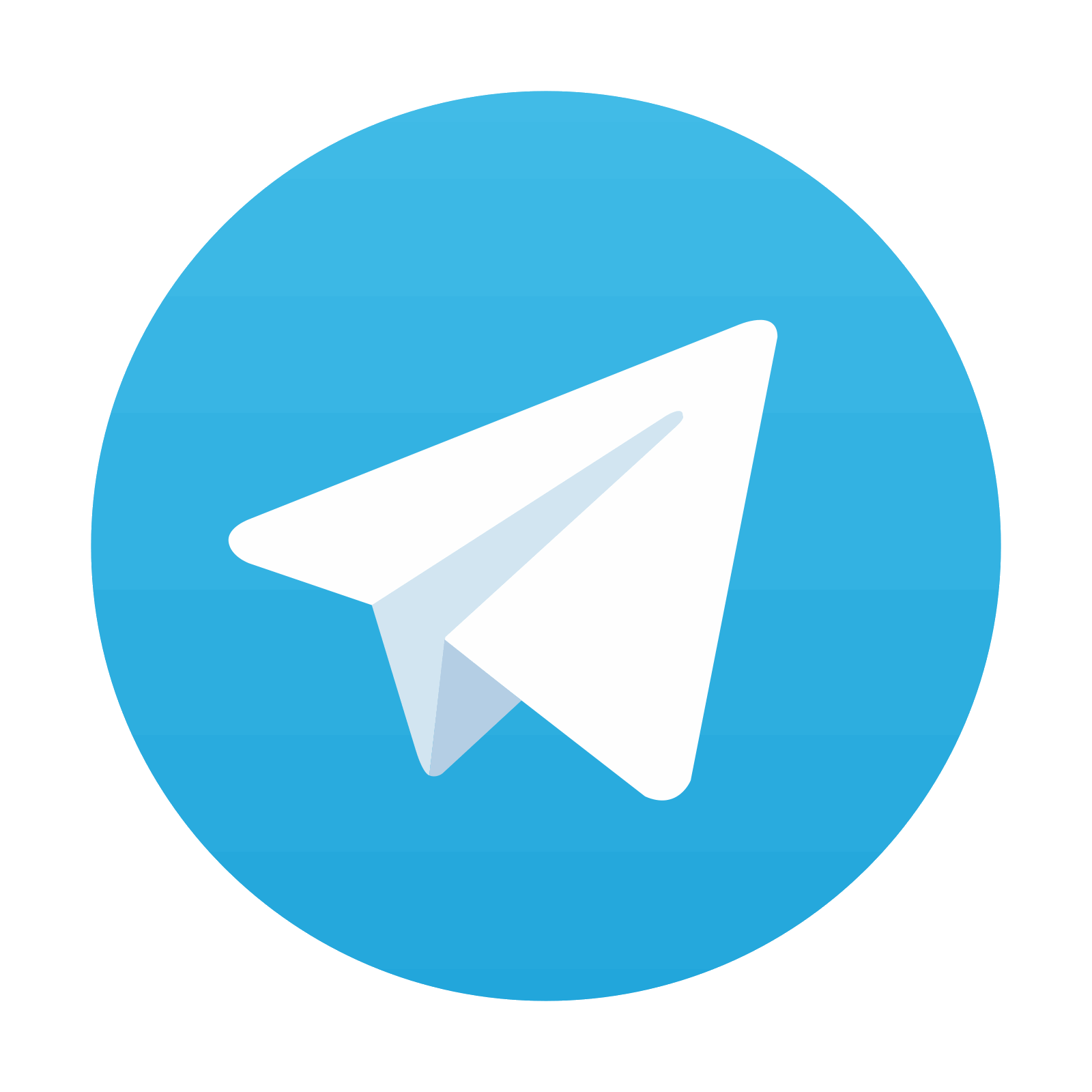
Stay updated, free articles. Join our Telegram channel
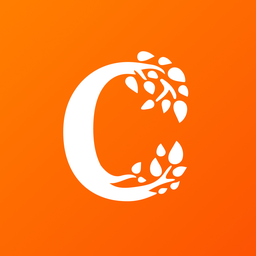
Full access? Get Clinical Tree
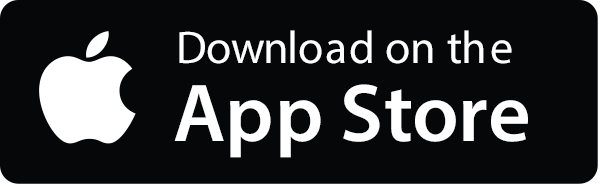
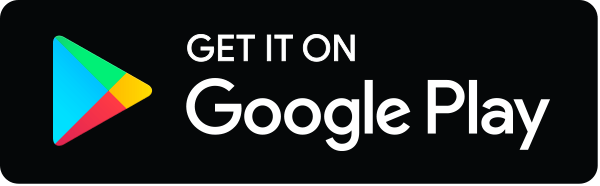
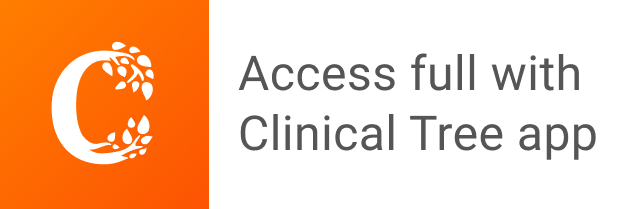