Vitreous Substitutes
Ivan J. Suñer
Mark E. Hammer
Vitreous substitutes have become an integral component of modern vitreoretinal surgery. They are primarily used as intraocular tamponades in retinal detachment procedures. However, they are also useful in stabilizing the retina during intraoperative maneuvers in complex vitreoretinal procedures, as well as in the intraoperative drainage of subretinal fluid.
BRIEF HISTORICAL OVERVIEW
In 1895, Deutschmann injected rabbit vitreous into the vitreous of a human to successfully treat retinal detachment.1 Intraocular air was first used for treatment of retinal detachment by Ohm in 1911.; Rosengren revived its use in 1938. Shortly thereafter, the use of intraocular air and gas for retinal reattachment declined with the advent of Gonin’s discovery that scleral buckling or indentation beneath the retinal break resulted in a high success rate for retinal reattachment. In the 1950s, Cibis began using silicone oil as a dissecting tool for proliferative vitreoretinopathy (PVR) detachments and also noted its tamponading effect. The development and application of other vitreous substitutes grew with the discovery by Kasner that the eye would tolerate fairly complete removal of the vitreous body and with the birth and development of pars plana vitrectomy.3 Further development of vitreoretinal applications of silicone oil was delayed by the advent of vitrectomy surgery to directly address vitreoretinal traction. As use of the vitrectomy procedure increased, aqueous substitutes for infusion during lengthy vitrectomy were developed. Long-acting gases for ocular tamponade after vitrectomy were developed in the 1970s.
Although silicone oil was never completely abandoned, it became much more popular for end stage detachments not responding to vitrectomy and tamponade with long-acting gases. In the 1980s heavier-than-water immiscible perfluorocarbon liquids were investigated for tamponade of inferior retinal tears and reattachment of the retina, especially for giant retinal tears. In 2001, semifluorinated alkanes were developed that were heavier than water and immiscible but possessed a lesser specific gravity than the perfluorocarbons, which allowed mechanical rotation of the partially flattened retina without gross mechanical damage to the retina.
A classification of vitreous substitutes based on their physical properties allows us to subdivide them into aqueous miscible vitreous substitutes (such as balanced salt solution, hyaluronic acid solutions, and other viscoelastic substances) and aqueous immiscible substances such as air, gases, fluorinated silicone oils, and perfluorocarbon liquids. The immiscible substances depend on their surface tension to seal retinal breaks either temporarily or on a long-term basis. They can be subdivided into gases (such as air, sulfur hexafluoride, perfluoropropane, and many other less frequently used gases) and liquids, including those that are lighter than water (polydimethylsiloxane, which we shall refer to as silicone oil) and those heavier than water (such as fluorinated silicone oils, perfluorocarbon liquids, and semifluorinated alkanes) (Table 54-1).
Table 54-1 Classification of Vitreous Substitutes | ||||||||||||||||||
---|---|---|---|---|---|---|---|---|---|---|---|---|---|---|---|---|---|---|
|
Several key concepts are important for a thorough and practical understanding of each of these vitreous substitutes. The mode of action and physical properties such as surface tension and viscosity must be considered. Biocompatibility, including direct toxicity, immune response, inflammatory response, and, in some cases, foreign body response, is important. The absorption or necessity for removal, indications for use (including clinical studies and special surgical methods), additives, and complications of use must also be considered.
Manufacturers of these vitreous substitutes and brand names when they exist are listed in this chapter. Many are still undergoing investigation by the U.S. Food and Drug Administration (FDA). The purity, quality, and cost of these vitreous substitutes are also important considerations.
AQUEOUS VITREOUS SUBSTITUTES
The perfect vitreous substitute would duplicate human vitreous with all of its hyaluronic acid (sodium hyaluronate), collagen fibrils, and electrolytes. This seems an unlikely achievement even in the distant future. In the stable postvitrectomy unicameral eye months after surgery, the vitreous component is likely to resemble that of aqueous, because the bulk of the fluid entering the eye originates from the ciliary body. From clinical experience, we know that eyes containing this aqueous secretion can survive indefinitely with good corneal and retinal function. For the present time, the ideal aqueous vitreous substitute would then approximate the human aqueous secretion.
The present vitreous substitutes used as infusion fluid during a vitrectomy approximate the aqueous humor. These infusions evolved from ocular irrigation fluids used during anterior segment surgery. Initially, normal saline was used, but corneal edema was found after even brief irrigation with this solution. Small volumes of a more finely tuned fluid called balanced salt solution (BSS) were then developed and were adequate for the relatively short procedures. In the 1970s, longer procedures such as vitrectomy, phacoemulsification, and anterior segment reconstruction developed. Larger solution volumes were required for these procedures. Plasma-Lyte 148 and lactated Ringer solution were used, but they resulted in significant postoperative corneal edema.4 BSS was superior to these two agents, but it still resulted in corneal edema and electroretinographic changes in surgeries lasting more than 1 hour.5 It was discovered that by fortifying BSS with bicarbonate, glucose, adenosine, and glutathione, corneal endothelial integrity and electroretinographic changes could be maintained, and retinal edema could be minimized in operations lasting longer than 1 hour.5,6 In the 1980s, BSS Plus became commercially available. Reduced glutathione is substituted for adenosine and oxidized glutathione, but otherwise BSS Plus is quite similar to the glutathione bicarbonate Ringer solution. To have a commercially acceptable shelf life, BSS Plus comes in a two-part solution and must be mixed at the time of surgery.
Sodium is the major extracellular ion required for maintenance of cellular tonicity and volume regulation of cells.7 Low potassium ion concentration (on the order of 3–6 mOsm/liter) is required to maintain the transmembrane cellular potential. Magnesium is necessary for many cellular biochemical reactions in all cells. Absence of the calcium ion during prolonged surgery will result in corneal edema caused by endothelial junctional breakdown.8 Calcium is also important in retinal functioning as well as retinal adhesion.9 Bicarbonate ion is a natural extracellular buffer that is important for both corneal functioning and maintenance of the electroretinogram during prolonged surgery. The bicarbonate ion is necessary for specific adenosine triphosphatases essential to intracellular functioning.10,11,12 Citrate, phosphate, N-2-hydroxyethyl-piperazine-N-2-ethane-sufonic acid (HEPES), and other buffers have been tried but are not adequate substitutes for bicarbonate ion.
Glutathione, which is metabolized by adenosine triphosphate for energy, is important in maintaining the clarity of the lens and the cornea and also retinal function during longer procedures. Glutathione has been shown to be helpful in maintaining corneal integrity in procedures lasting up to 6 hours. Although glutathione can be helpful in maintaining intracellular adenosine triphosphate, glucose alone is capable of accomplishing this. The unique feature of glutathione is that it may detoxify injurious free radicals generated during intraocular surgery.13 Ascorbate, which is a naturally occurring antioxidant in aqueous fluid, may protect against retinal-pigmented epithelial light damage.14
The cornea has been shown to tolerate osmolalities ranging between 200 and 400 mOsm, but the osmolality of normal anterior chamber fluid is 305 mOsm. It is best to have a fluid of normal osmolality because in many surgical cases, the corneal epithelium is already compromised. The pH range tolerated by the cornea has been shown to be 6.8 to 8.2. Because the pH of the normal anterior chamber fluid is 7.4, ocular infusion fluid is usually adjusted to a pH of approximately 7.415 The pH is vulnerable to additions to the irrigating solution. Surgeons should be aware that additives to irrigating solution might create a pH value leading to lenticular, corneal, and retinal toxicity and compromise.16
Glucose has been used to fortify BSS Plus in phakic diabetic vitrectomy.17 It was found that posterior capsular opacities would develop during prolonged vitrectomy with BSS Plus.18 This decreases the risk of intraoperative lens opacification and need for lensectomy in diabetic persons, which may quadruple the risk of developing postoperative neovascular glaucoma in diabetics. Additional glucose is usually given in the form of injection of a small aliquot of 50% glucose into a 500-mL bottle of BSS Plus. This has the effect of increasing the osmolality of the infusion fluid. Lenses of a diabetic person have polyols trapped within them. When the vitreous fluid osmolality is lowered to 305 mOsm, a transcapular osmotic gradient tends to draw in extra fluid, creating an osmotic stress and a posterior subcapsular cataract. Fortified BSS Plus can be prepared by adding 3 mL of a 50% dextrose solution in sterile water with no preservatives to the standard 500 mL bottle of BSS Plus.19 This results in increasing the glucose concentration to between 300 and 400 mg/mL and gives a pH of 7.5 and an osmolality of approximately 320 mOsm. In an animal model, up to 6 mL of 50% glucose has been added, yielding an osmolality of 330 mOsm and a pH of 7.5. The resulting solution had a concentration of 650 to 750 mg/dL. One series using a fortified glucose solution cited only two occurrences of posterior subcapsular lens changes in 1,000 vitrectomy cases (Table 54-2).20 Epinephrine is frequently added to BSS Plus infusion solutions to maintain pupillary mydriasis during prolonged surgery (Table 54-2).21 In undiluted and weakly diluted solutions, the bisulfite preservative included in most epinephrine preparations is shown to cause corneal endothelial damage and subsequent corneal haziness.22 The corneal damage, however, can be prevented by diluting bisulfite-containing solutions to 1:5,000 or greater or by actually preparing an epinephrine bitartrate solution at 1:1,000 concentration.23 It has been shown that extremely diluted concentrations of epinephrine (on the order of 1:96,000 or less) are effective in maintaining mydriasis during intraocular surgery. In practice, 0.3 to 0.5 mL of 1:1,000 epinephrine is usually added to a 500-mL bottle of BSS Plus for vitrectomy surgery. Concentrations of 1:1,000 Parke Davis epinephrine and 1:1,000 Elkin Sinn epinephrine in the amount of 1 mL to 500 mL of BSS Plus produced no toxic effects on endothelial tissue.
Table 54-2 Common Additives to Vitreous Irrigating Solution | |||||||||
---|---|---|---|---|---|---|---|---|---|
|
Other additives such as atropine, chymotrypsin, acetylcholine, and carbachol have specifically been demonstrated to have low corneal endothelial toxicity when used in diluted solution.24,25 These are not in general usage or certainly are used infrequently by vitreoretinal surgeons. Dilute solutions of bovine thrombin have been used to prevent hemorrhage during vitrectomy for diabetic retinopathy but were abandoned after frequent inflammatory and fibrinous responses occurred, possibly related to the nonhuman source of the thrombin. Dilute solutions of heparin were also used but again found not to have significant advantage in controlling hemorrhage during vitrectomy. Antibiotics placed in the infusion fluids have been used by some surgeons to decrease the risk of endophthalmitis. Use of dextran has been suggested by some vitrectomists to dehydrate the cornea, but this is not in wide usage.
It has been suggested that hypothermia of ocular fluids protects the retina from light toxicity during vitrectomy.26 Retinal adhesiveness is increased by hypothermia. In a sense, infusion fluids are usually relatively hypothermic because they are administered at room temperature, which is considerably below body temperature. Some attempts have been made to further cool intraocular fluids during vitrectomy, but cooling is not widely used at this time.
Retinal adhesion both postmortem and in vivo has been shown to be affected by several factors in infusion fluids. For example, low calcium ion concentration weakens retinal adhesions significantly. This may be applicable to newer techniques that require the surgical creation of retinal detachments, such as macular rotation surgery. Factors that increased retinal adhesion are ouabain, hyperosmolar solution, and darkness rather than light.27 Factors weakening retinal adhesion were normal saline, low pH, and solutions without calcium. There was little effect on retinal adhesion by removing magnesium, potassium, sulfate, and orthophosphate.28 These factors require more investigation before clinical application.
VISCOELASTIC SUBSTANCES AS VITREOUS SUBSTITUTES
Use of hyaluronic acid as a vitreous substitute was first proposed by Balazs in 1960. The development of hyaluronic acid has been most successful in anterior segment surgery. It was first used in lens implant surgery in 1979. Fechner used methylcellulose 1% solution to coat intraocular implants in 1977, and in the 1980s, it was used for the maintenance of anterior chamber depth and stability. A combination of chondroitin sulfate and hyaluronic acid for use as an anterior segment viscoelastic substance became available in the 1980s. The vitreous surgeon uses viscoelastic substances to maintain the clarity and integrity of the anterior segment during vitrectomy surgery. Many important posterior segment uses of viscoelastic substances have developed mainly around hyaluronic acid. Except in a few special circumstances, viscoelastic substances are not used as bulk or volume vitreous substitutes.
The relatively high viscosity or resistance to flow of these commercially available viscous agents is their most striking characteristic. At the molecular level, viscosity arises from the interactions of relatively large molecules as their concentration increases. Pseudoplasticity is an important concept that explains how some of the relatively thick solutions can be extruded through a small-gauge cannula.29 A pseudoplastic fluid is one in which the viscosity actually decreases as the shear rate of the fluid increases.30,31 The relationship of shear rate to flow through the needle can be understood by visualizing the velocity profile of the fluid as it flows through the lumen of the needle. At the wall of the needle, the velocity is zero. In the center of the fluid, velocity is maximum. In between a parabolic curve represents the velocity, which is zero at each wall and maximal at the center of the needle. The tangent to this curve, which is the slope at any given distance, x, across the lumen of the needle, is the shear rate and is expressed as dv/dx (Fig. 54-1). Thus, the shear rate is much higher, and the apparent viscosity is vastly lower near the wall of the needle for extremely pseudoplastic fluids such as hyaluronic acid. The vastly lower viscosity near the wall of the needle decreases the resistance to flow enormously. This allows the pseudoplastic fluid in the central channel of the needle to flow almost as a plug. This explains the paradox that concentrated hyaluronic acid has high apparent viscosity at rest but can still be injected through a 30-gauge needle. The pluglike flow through a small-diameter needle also explains the extruded appearance of the hyaluronic acid from the end of the needle. Hyaluronic acid at a 1% concentration is the most pseudoplastic of the commercially available substances and therefore can be injected with 27- to 30-gauge needles. Hydroxypropyl methylcellulose (HPMC) is less pseudoplastic and requires a larger (23–25) gauge needle for injection.
Another important concept is the Reynolds number, the dimensionless number that can be used to determine whether a fluid system is more likely or less likely to develop a turbulent or rough fluid flow. Fluid systems that have a Reynolds number greater than 2,000 may develop turbulent flow, whereas those with a Reynolds number less than 2,000 generally will not. The Reynolds number is equal to the characteristic velocity of fluid, multiplied by a characteristic length, multiplied by the fluid density, and divided by its viscosity. An eye filled with BSS has a Reynolds number of around 5,700 (Table 54-3). When 0.4 mL of 1% hyaluronic acid is added, the Reynolds number is reduced to approximately 250 once the hyaluronic acid goes into a uniform solution in the vitreous. Thus, relatively small amounts of hyaluronic acid in the posterior segment can result in reducing turbulent and rough fluid motions at the retinal surface.

Table 54-3 Reynolds Number | ||||||||||||||
---|---|---|---|---|---|---|---|---|---|---|---|---|---|---|
|
The viscosity of hyaluronic acid/sodium hyaluronate (Healon) when it is not moving or is at zero shear is as high as 300,000 centipoise (cP), but viscosity falls to around 200 cP at shear rates of 1,000 inverse seconds. Viscoat is a hyaluronic acid and chondroitin sulfate mixture that has a viscosity of approximately 40,000 cP at rest and of approximately 300 cP at a shear rate of 1,000 inverse seconds. The viscosity of Amvisc parallels that of Healon but appears to be less at very low shear rates. The viscosity of Amvisc Plus appears to be slightly greater than that of Healon at very low or zero shear rates. The zero shear rate viscosity predicts the ability of the viscoelastic substance to maintain the anterior chamber depth. The viscosity of Occucoat is 4,000 cP at zero shear and about 300 cP at 1,000 inverse seconds. Occucoat, a commercially available HPMC, shows less pseudoplasticity.
The hyaluronic acid and chondroitin sulfate substance has a greater affinity to coat instruments and stick to tissues than 1% hyaluronate.32 This is usually attributed to the fact that the hyaluronate–chondroitin complex carries three negative charges for every one that the hyaluronate molecule carries. HPMC solutions also have good coating properties. The pH of commercially available solutions is in the range of 6.5 to 7.5; it should be adjusted to a pH of 7.4 wherever possible. The osmolality of commercially available viscous solutions ranges from 285 to 340 mOsm/kg H2O. Chondroitin sulfate was developed as a viscous solution; however, its osmolality is 1,000 mOsm in a 50% solution, which is required for higher viscosity. This is damaging to the corneal endothelium.
The FDA approved viscoelastic substances that are nontoxic to the ocular tissues (Table 54-4). The substances must be highly purified to prevent inflammatory reactions, presumably of a foreign antigen type. HPMC solutions can be autoclaved without damaging their molecular structure, but hyaluronate solutions must not be autoclaved. Autoclaving and reuse of cannulas not properly cleaned after hyaluronic acid injection has been thought to lead to inflammatory reactions in subsequent operations. Viscoat, the commercially available chondroitin sulfate and hyaluronate solution, was reformulated in 1987 to contain a smaller amount of phosphate buffer. The higher phosphate concentration in the earlier formulations led to the precipitation of calcium in the cornea, seen as an acute band keratopathy.33 This was noted to occur in association with the use of BSS Plus but not BSS, which contains a citrate buffer that probably prevented potassium precipitation by high amounts of phosphate.
Table 54-4 Properties of Viscoelastic Substances | ||||||||||||||||||||||||||||||||||||
---|---|---|---|---|---|---|---|---|---|---|---|---|---|---|---|---|---|---|---|---|---|---|---|---|---|---|---|---|---|---|---|---|---|---|---|---|
|
When injected into the unicameral, eye the viscoelastic solutions leave the eye through the trabecular meshwork. Hyaluronic acid injected into the vitreous probably also leaves through the trabecular meshwork, but it takes a great deal longer. In the owl monkey, hyaluronic acid flows out of the anterior chamber within 72 hours. When injected into the vitreous of the phakic eye, however, it may take more than 2 months to flow out. There are no reports of commercially available chondroitin sulfate or HPMC solutions used in the posterior segment of aphakic eyes. Early reports of white precipitates and inflammation occurring after HPMC injection into the posterior segment of rabbits were based on laboratory rather than commercial preparations of HPMC.34 These reactions may have resulted from inadequate purification. Vegetable fibers and other impurities were found in HPMC preparations made by pharmacies from non–medical-grade products. The human body has enzymes to degrade hyaluronate and chondroitin sulfate, but hyaluronate at least is not degraded within the eye. HPMC cannot be completely degraded by any human enzymes. Chondroitin sulfate has induced epiretinal membranes when injected into the rabbit vitreous.35 Although the purity of the chondroitin sulfate was carefully evaluated, the investigators did not use a commercially available, FDA-approved combined chondroitin sulfate and hyaluronate solution.
Surgical removal of viscoelastic solutions is recommended in anterior segment surgery because of high postoperative intraocular pressures that presumably result from occlusion of the trabecular meshwork by large molecules of the viscoelastic solution.36 High and sometimes uncontrollable pressure spikes occurring after the use of intraocular viscoelastic solutions are the most troublesome complication.37 The use of large amounts of hyaluronate in the posterior segment can cause very high intraocular pressure, requiring a second operation to remove the viscoelastic substance.38 Meticulous removal of hyaluronic acid at the end of most posterior segment applications is not usually done and, indeed, could be as challenging as the associated vitreoretinal surgery. High intraocular pressures are much less frequently noted with posterior injection of viscoelastic solutions in phakic rather than aphakic eyes. The chondroitin sulfate and hyaluronate solution is less likely to give increased intraocular pressures and may give more easily controlled pressure spikes than hyaluronic acid.39 The lowering of the pressure spike is most pronounced when the chondroitin and hyaluronate solution is aspirated at the end of surgery. This is due to the lower molecular weight of chondroitin sulfate and hyaluronate in Viscoat relative to the hyaluronic acid in Healon or Amvisc. When unacceptable pressure elevations occur, treatment with acetazolamide, β-blockers, and 4% pilocarpine gel have been shown to be effective in reducing postoperative intraocular pressure. If these efforts fail, aspiration or irrigation of the remaining viscoelastic solution is indicated. This is particularly relevant in posterior segment surgery, when large amounts of viscoelastic substance may remain in the eye for very long periods of time.
Viscous vitreous substitutes are used extensively by anterior segment surgeons in extracapsular cataract extraction, phacoemulsification, lens insertion and removal, and corneal transplantation. The most common use of viscoelastic agents by the vitreoretinal surgeon is as an optical binding substance between the corneal contact lens and the corneal surface. All three major viscoelastic substances are effective for this use. Viscoelastic solutions placed on the corneal surface during a long retinal procedure slow down corneal epithelial desiccation and decrease the frequency of wetting required. This is especially helpful in the use of the noncontact binocular indirect ophthalmoscope in conjunction with the stereo diagonal inverter. Here, the objective lens of the system can be quite close to the cornea. Frequent wetting of the cornea may splash droplets onto the objective lens surface. Using a viscoelastic solution decreases the frequency of corneal wetting so visualization is less frequently compromised. HPMC solutions are preferable to hyaluronate solutions in this instance because they are less viscous and less elastic, and replicate the corneal curvature much more rapidly than concentrated hyaluronic acid solutions. This is important because the concentrated hyaluronic acid often develops its own contour, which results in irregular astigmatism, causing either a blurred image or a distorted image through the microscope.
Viscoelastic solutions are frequently used in the anterior chamber when an intraocular lens is removed or placed by the vitreoretinal surgeon. All of the viscoelastic solutions appear to give good protection of the corneal endothelium from potential lens touch during insertion or removal of an intraocular lens. In cases in which the iris has become adherent to the corneal epithelium over fairly wide areas, the use of concentrated hyaluronic acid solutions is perhaps the gentlest method of dissection and offers the best hope of retaining corneal endothelial function.
Viscoelastic solutions such as hyaluronic acid are valuable as viscous agents in repair of corneal lacerations during posterior segment surgery. Hyaluronic acid solutions are the most effective for maintaining an optically clear plug in the anterior chamber when oozing blood is trapped there by either an intraocular or a native lens. The surgeon should remove at least some of the viscous material from the anterior chamber at the end of the surgery to minimize the risk of a postoperative pressure spike. A similar technique is useful in vitrectomy for endophthalmitis, in which continued oozing and settling of white blood cells and fibrin trapped by an intraocular lens may prevent good visualization during vitrectomy.
Hyaluronic acid is frequently used on the posterior corneal surface as an aid to visualization after fluid–gas exchange.40 After a long procedure, the cornea may have multiple folds in a Descemet membrane. When fluid–gas exchange is performed, the folds in the Descemet membrane at the fluid–air interface can cause pronounced image degradation and may prevent further surgery. Coating the posterior surface of the cornea with a hyaluronic acid solution will improve the image so that surgery can be completed.41 Theoretically, HPMC and combined hyaluronate and chondroitin sulfate solutions could be superior to hyaluronate solutions alone because they would tend to form a smoother fluid viscoelastic substance interface more quickly than hyaluronate solution. There are, however, no reports in the literature of the use of these two solutions for such a purpose.
There has been some suggestion by anterior segment surgeons that viscoelastic solutions may protect the corneal endothelium from high flow and turbulence during phacoemulsification.42 Controlled animal experiments, however, have shown no benefit of hyaluronic acid or the hyaluronate–chondroitin sulfate mixtures over BSS Plus in protecting the corneal endothelium.43 There is no compelling reason for the vitreous surgeon to use such a solution during ultrasonic lensectomy at this time.
Hyaluronic acid solution has been used as a bulk vitreous substitute in the vitreous cavity and to replace volume as choroidal hemorrhagic detachments are drained. A single case is reported. There was no abnormal rise in intraocular pressure postoperatively.44 A good visual recovery was also achieved.
Concentrated hyaluronic acid solution has been injected in the suprachoroidal space to create a temporary scleral buckle.45 This was used to treat both recurrent detachments after scleral buckling and primary detachments. The hyaluronic acid formed an adequate buckle for approximately 1 week, then slowly reabsorbed over the second week. One case was complicated by development of spontaneous nonhemorrhagic choroidal detachments, but there was no report of hemorrhagic choroidal detachment. Fourteen retinas were reattached with at least 5 months of follow-up. Hyaluronate solution, 0.3 to 0.8 mL, was injected through a sclerotomy down to the level of the choroid. Subretinal fluid was drained before injection in 12 of the patients.
Healon has been used as a bulk vitreous substitute in retinal detachment surgery after drainage of subretinal fluid in the same way that BSS and intraocular air or gas are used to restore normal ocular tension.46 This is usually done in conjunction with scleral buckling and cryopexy or laser treatment.47 Although the hyaluronic acid solution had excellent biocompatibility, Koster and Stilma48 found at least a transient pressure rise in 19 of 40 patients. Vatne and Syrdalen38 found intraocular pressure rises of 60 to 70 mm Hg, which necessitated removal of concentrated hyaluronic acid solution to control the intraocular pressure. In this study, the hyaluronic acid actually passed through a retinal break into the subretinal space and was assessed to be mechanically preventing retinal reattachment because of a slow resorption of the viscous substance from the subretinal space. This study, however, dealt mainly with patients with PVR grades C and D. It was concluded that hyaluronic acid solutions are at best a limited addition to conventional scleral buckling surgery in PVR detachment. A relatively large amount of hyaluronic acid solution is required for bulk vitreous substitution, creating a fairly substantial expense.
Hyaluronic acid solutions have been used to unfold giant tears, especially those with rolled-over flaps.49 This technique had been moderately popular but has been supplanted by the use of liquid perfluorocarbons (discussed further in that section).
Elevation and partial dissection of preretinal membranes with injection of viscoelastic substance with a small cannula has been advocated.50 Such surgery has been performed with both hyaluronic acid solutions and in one case with HPMC. The much higher viscosity of hyaluronate as well as its ability to be injected through a cannula as small as 30 gauge would seem to confer a significant advantage. Increasing the viscosity of Healon51 and tinting the Healon with fluorescein have facilitated the procedure.52 Experience with this technique has been that it dissects the membranes nicely where they are loosely adherent. Loosely adherent membranes, however, can be easily removed without the use of viscous dissection. In the more difficult and tightly adherent membranes, the viscous dissection does not work very well. Furthermore, the hyaluronic acid solution adheres to the tissues of interest. This lubricates them and makes them hard to grasp with instruments. The solution is difficult to remove and disrupts the local hydrodynamic flow with the vitrectomy device and aspirating suction. In vitrectomy for advanced cicatricial retinopathy of prematurity, hyaluronic acid has been advocated as a volume vitreous substitute to hold open the funnel extending posterior to the optic nerve during dissection.
The procoagulant affects of concentrated hyaluronic acid solutions after phakic diabetic vitrectomy have been investigated. In a prospective randomized study, rebleeding was significantly reduced with injection of 0.5 to 0.75 mm of hyaluronic acid at the end of phakic diabetic vitrectomies.53 At 2 weeks, the amount of time in which the hyaluronic solution concentration would be effectively reduced, the procoagulant effect disappeared. In a previous study, only one of four aphakic diabetic eyes with severely increased intraocular pressure could be controlled with timolol and acetazolamide. The pressures of these patients ran in the 40- to 50-mm Hg range for 3 to 6 weeks. Because all of these eyes were thought to have a very poor prognosis preoperatively, removal of hyaluronic acid was not performed. The authors were unable to find any anticoagulant effect and concluded that hyaluronic acid forms a barrier that actually encases small bleeding sites. Additionally, the increased viscosity caused by hyaluronic acid in the posterior segment could decrease turbulent flow over newly formed clots. The basis for this is noted in the previous discussion of the Reynolds number (Fig. 54-2).
INTRAOCULAR GASES AS VITREOUS SUBSTITUTES
The first use of intraocular air recorded in literature was in 1911 by Ohm.2 He and several subsequent investigators were unaware of the relationship of retinal holes and retinal detachments, but they reported cases in which they successfully reattached the retina. Subsequent to Gonin’s discovery that retinal holes were the cause of retinal detachment, Rosengren used intravitreal air injection with drainage of subretinal fluid and diathermy. He achieved a 77% reattachment rate, which was a tremendous advance at that time. In the 1950s, the use of air became less popular due to rapid advances in scleral buckling techniques. Intravitreal air was used as an adjunct to buckling procedures throughout that period. In 1973, Norton54 used sulfur hexafluoride (SF6) gas for a longer tamponade than was provided by air injection for difficult retinal detachments, particularly giant tears.55 Vygantas et al56 were the first to use perfluorocarbon gases to provide a longer intraocular tamponade than SF6. Lincoff 57 then developed several perfluorocarbon gases. Air, SF6, and perfluoropropane were used for many new vitrectomy procedures throughout the 1970s and 1980s. In 1984, Hilton and Grizzard58 reported a series of pneumatic retinopexy patients in which they advocated the use of intraocular gas tamponade as a primary treatment for selected retinal detachments.58
MODE OF ACTION AND PHYSICAL PROPERTIES
Surface tension between gas bubbles and surrounding fluids is the most important physical property of the gases in retinal reattachment. The gas bubble is positioned so that it occludes the retinal break either continuously or a substantial percentage of the time to prevent bulk flow of fluid through the defect. This allows the natural reabsorption of subretinal fluid to slowly reattach the retina. Surface tension arises from electrostatic attractive forces between fluid molecules. These van der Waals forces are both weaker and longer range than the electronic exchange forces of the chemical bond. Van der Waals forces are responsible for condensation of vapors into liquids. The most important attractive force is the dispersion or London-van der Waals force, which arises from neutral atoms because they are oscillating systems of negative electronic charges around a positive nuclear charge.59
Hydrogen bonding and dipolar interactions also can play a role in surface tension. Dipolar forces arise from uneven charge distribution around a molecule. Internal molecular attraction forces neutralize each other in the bulk of the fluid but are not neutralized at the surface. The surface tension arises from lateral extension of the surface caused by crowding of interior molecules being brought to the surface by the attractive forces that are not neutralized. The attractive intermolecular forces decrease as the seventh power of the intermolecular distance. Thus, the surface tension layer is only one to two molecules thick. The saline–gas interface produces the highest surface tension available to the ophthalmologist (measuring approximately 60 ergs/cm2), as the attractive forces in the saline are not neutralized by the overlying air at the air–fluid interface.
Another important property of gas is buoyancy, which is a result of the large difference in specific gravity between fluid and gas. A fresh, freely movable superior tear tamponaded with a large air bubble will usually displace fluid inferiorly and away from the tear, allowing it to flatten against the wall of the eye. Buoyancy directs the effectiveness of the tamponade, which for gases on earth must always be in the gravitational upward direction. Large bubbles and face-down positioning are required to tamponade inferior retinal breaks.
The solubility of a gas in the aqueous medium is the most important property determining the reabsorption rate of a gas bubble from the vitreous cavity.60 If the gas bubble is less soluble than nitrogen, expansion of the bubble can occur. Expansion can also occur with relatively soluble gases such as air when the patient is breathing nitrous oxide, which is more soluble than air in water.
The relationship between bubble volume and its arc of contact inside the eye is important. It determines how much gas is necessary to treat a tear or group of tears of a specific size and proximity. By using a glass model eye, it was determined that in an average eye with a diameter of 21 mm, approximately 0.28 mL of gas is required to give a 90° arc of contact.61 In a larger myopic eye of 24-mm diameter, 0.42 mL would be required. The respective volumes for an arc of contact of 120° are 0.75 mL for the normal eye and 1.13 mL for the myopic eye. An arc of contact of 180° requires respective bubble sizes of 2.4 mL and 3.62 mL. A later study using computed tomography of actual human eyes showed that for an arc of contact of 90°, a somewhat greater volume of approximately 1.5 mL was required (Fig. 54-3).
BIOCOMPATIBILITY
SF6 and the perfluorocarbon gases have a purity of 99.8% or better. Although they are frequently injected as mixtures with air, the pure gases are usually described as chemically nonreactive, colorless, odorless, and nontoxic. Earlier papers show haziness of the vitreous after SF6, perfluorocarbon, and air injection.62 Pathologic examination of the retina showed some changes in the outer layer of the retina, but these changes were no worse in eyes injected with long-acting gas than in the eyes injected with air.63 A study using SF6 and perfluoropropane monitored by the FDA under an investigational device exemption for pneumatic retinopexy showed no significant flare or cell with the biomicroscope.64 No significant vitreous inflammation measured by the National Eye Institute scale was noted for either SF6 or perfluoropropane. SF6 may contain up to 0.3 ppm of hydrogen fluoride. This is generally regarded as the most toxic contaminant found in SF6. There is an established threshold limit value for hydrogen fluoride in the workplace. Similarly, perfluoropropane may contain up to 10 ppm of perfluoropropylene. Perfluoropropylene is a toxic gas for which a threshold-limiting value has also been established. Because of the stricter FDA standards, modern gases may contain less of these contaminants than gases used by early investigators. Greater purity, smaller injection volumes, and more attention to conjunctival sterilization could explain why recent investigators have seen a quiet vitreous, whereas earlier investigators observed the flare and cell or a hazy vitreous response. If the bubble is large enough to cover the back of the lens, a cataract will develop unless the patient is positioned so that a layer of fluid covers the posterior surface of the lens.65 This is thought to result from a drying or deprivation of nutrient effect rather than from a toxic effect of the gases.
Prolonged contact with the corneal endothelium has been shown to cause increased inflammation, seen to a greater extent with SF6 than perfluoropropane.66 Both gases caused persistent corneal edema and posterior corneal membrane formation. Posterior corneal membrane formation has been described in more than 20 disorders and is due to interference with nutrition of the endothelium rather than to a specific toxic effect.
ABSORPTION AND KINETICS OF GASES
Intraocular gas bubbles are eventually reabsorbed. Inert gases such as helium, neon, argon, krypton, and xenon are reabsorbed more rapidly than air. Thus they are nonexpansile gases when breathing air. Nitrous oxide is extremely soluble in blood and tissue, and can lead to significant expansion of even an air bubble during the course of an operation. Breathing 100% oxygen and a volatile anesthetic, however, causes little change in an intraocular gas bubble during anesthesia.
The kinetics of the less water-soluble gases such as SF6 and perfluorocarbon are much more complex and have been studied in substantial detail.67,68,69,70,71,72,73 Injection of a bubble of 100% SF6 or perfluoropropane leads to expansion of the bubble. The volume continues to increase, reaching a maximum after 2 to 4 days for SF6 and 4 to 5 days for perfluoropropane. The main constituent gas that leads to expansion of the gas bubble is nitrogen. About 10% of the gas bubble concentration is carbon dioxide and oxygen, which remain at a fairly constant low concentration throughout the life of the gas bubble. Eventually, the partial pressure of nitrogen in the bubble equilibrates roughly with the nitrogen partial pressure in the capillary blood. The proportions of gases in the bubble then remain constant as the bubble volume gradually decreases and all the gases leave the eye at the same rate. Because the long-acting gas is the slowest to leave the eye, it essentially controls the rate of clearance of the bubble volume in the final stage.
This dynamic has been summarized as having three phases (Fig. 54-4). The first phase is bubble expansion caused by rapid diffusion of nitrogen into the bubble. The second phase lasts from maximum expansion of the bubble until the nitrogen concentration in the bubble is stable and roughly the same as its concentration in venous blood. The third phase is from the time of nitrogen equilibration until the bubble is reabsorbed.
The clearance of gas in the third stage is approximately described as a first-order exponential equation having the form V = V0ekt, where V0 is the volume at the time of equilibration of nitrogen concentration with that in the blood and V is the volume at any subsequent time, t. K is a constant for a particular gas. A half-life for the bubble determining the time it takes for V to equal one half of V0 can be calculated once k is determined. Perfluoromethane, perfluoroethane, and perfluoro-n-butane also have an exponential decay pattern. This description must be regarded as approximate because it would imply that small bubbles would just get smaller but never really disappear. This is contrary to observation because smaller bubbles are noted to disappear rather abruptly. The half-life of the disappearance phase of SF6 has been reported to be 2.4 to 2.8 days and that of perfluoropropane to be 4.5 to 6 days. The gases are removed from the eye more rapidly when the eye is either aphakic or pseudophakic. The bubble lasts much longer in the presence of severe hypotony. Many publications indicate the average time to disappearance of bubbles of various size and of various gas mixtures with air injected into the vitreous. From clinical and animal observations, the actual time of disappearance depends on concentration of the gas, the size of the gas bubble injected, whether the patient is aphakic or phakic, and whether there is a hypotony. The theoretical factors governing gas leaving the eye are the molecular weight of the gas, diffusion coefficient of tissues, water solubility of the gas, intraocular pressure, inflow rate of ciliary body secretion, phakic or aphakic status, hypotony, vitrectomy, stirring of vitreous fluid by ocular motion, bulk outflow of aqueous through trabecular meshwork, posterior choroid venous concentration of gases, and vascular perfusion rate of the choroid. In most models, the most important factors seem to be the water solubility of the gas, hypotony, aphakia, vitrectomy, and bulk flow of water from the eye. Theoretical models show reasonable approximate results but are too complex to accurately predict what will happen for an individual patient. Certainly, determining the optimal size and composition of a gas bubble injected into a specific patient in a specific circumstance is part of the art of vitreoretinal surgery at this time.
One approach to gas injection is to estimate the maximum bubble size expected from the amount injected. Excessive volumes of pure expansile gases can lead to a maximum bubble size larger than the ocular volume. Extremely high pressures resulting in central retinal artery occlusion and loss of light perception then occur. One would also like to keep the maximum size of the gas bubble slightly less than vitreous volume to prevent cataract formation in phakic patients and corneal opacification in aphakic patients. Generally accepted estimated ratios of the maximum volume of the expanded gas bubble to the original volume of injected gas are 2:1 for SF6; 2:1 for perfluoromethane; 3.25:1 for perfluoroethane; 4:1 for perfluoropropane; and 5:1 for perfluoro-n-butane.
Another approach to the problem of an expanding gas bubble is to inject nonexpansile or a slightly expansile concentration of the gas. The nonexpansile concentration of SF6 was initially reported at 40%. Most surgeons err on the side of caution by using a 20% concentration of SF6 when attempting a total or near-total fluid–gas exchange at the conclusion of vitrectomy. A nonexpansile concentration of perfluoropropane is thought to be 12%.74 Surgeons frequently use concentrations between 10% and 16% perfluoropropane for large posterior segment tamponades.
In pneumatic retinopexy, in which relatively small volumes of gas are injected, pure gases can be safely used because they are unlikely to overfill the vitreous volume. An estimate has been made of the amount of time a therapeutic volume of gas is maintained by injection of 0.3 mL of gas. A therapeutic volume of gas for pneumatic retinopexy has a 90° arc of contact and will tamponade a break for approximately 1 clock hour. The amount of time estimated for the presence of therapeutic volume of gas is for air, less than 1 day; for SF6, 3 to 4 days; for perfluoromethane, 2 days; for perfluoropropane, 16 days; and for perfluoro-n-butane, 30 days.75 SF6 and perfluoropropane are the most common gases used in small-volume injection for pneumatic retinopexy. There are reports of the effective use of air, but this requires a greater volume.76 Volumes injected for pneumatic retinopexy usually vary between 0.3 and 0.6 mL (Table 54-5).
Table 54-5 Clinical Properties of Intraocular Gases | ||||||||||||||||||||||||||||||||||||||||||||||||
---|---|---|---|---|---|---|---|---|---|---|---|---|---|---|---|---|---|---|---|---|---|---|---|---|---|---|---|---|---|---|---|---|---|---|---|---|---|---|---|---|---|---|---|---|---|---|---|---|
|
INDICATIONS AND TECHNIQUES FOR INTRAOCULAR GASES
Interest in gases began when Norton et al55 used intravitreal air to unfold giant retinal tears that had a poor success rate with scleral buckling alone. Machemer and his associates then investigated SF6 with the aim of finding a longer tamponade than that given by air. They sought a higher success rate for giant retinal tears of greater than 180° with the longer tamponade. When the longer-acting gas was used in conjunction with newly developed vitrectomy, Machemer and Allen77 were able to reattach 12 of 14 cases with tears of 180° or greater. At 6-month follow-up, however, only 6 of the 14 cases remained attached because of the development of PVR. Lensectomy was a further improvement in management of giant retinal tears with large tears and PVR. Surgery on a special table with face-down positioning of the patient was required to unfold the retina and minimize posterior slippage of the retinal break. After air–fluid exchange, a thin layer of fluid remaining under the retina usually resulted in the retinal flap sliding posteriorly in the supine position. Using a cannulated extrusion needle, Joondeph et al78 removed subretinal fluid and brushed or held the retina anteriorly at the completion of air–fluid exchange. Posterior slippage was controlled with surgery in the supine position in 18 eyes. Perfluorocarbon liquids are now the most common method used to unfold retinal tears with the patient in the supine position. Silicone oil has been used as a long-term tamponade in cases of giant retinal tears. Although silicone oil gives superior results to SF6, in cases with PVR, no advantage over perfluoropropane has been proven. This factor is discussed in more detail in the section on silicone oil.
Treatment of detachments caused strictly by macular holes is successfully approached with intraocular gas bubbles.79,80 When the vitreous is separated from the posterior segment, vitrectomy is not required for lasting retinal reattachment. Strict face-down patient positioning is required. Because of the expansile properties of the perfluorocarbon gases, small-volume injections are made without requiring drainage of subretinal fluid. Vitrectomy can be used when vitreoretinal traction is present. The retina usually remains adherent after reattachment without use of laser treatment around the macular hole. In some difficult recurrent cases, laser treatment around the reattached retinal hole will maintain retinal reattachment. Face-down positioning was maintained for 8 to 11 days in tolerant patients. This was based on the notion that a firm adhesion between the neurosensory retina and the retinal pigment epithelium takes 8 to 11 days to form after treatment with laser or cryopexy.
The use of intraocular gases to tamponade the retina in PVR has become extremely important. There has been a suspicion that even SF6 did not provide a long enough tamponade to develop sufficient adhesion to counter the epiretinal, subretinal, and transvitreal traction forces of PVR. Perfluoropropane has been proven to give superior results in tamponading postvitrectomy PVR cases. Of 18 patients treated with vitrectomy and perfluoropropane, in the successfully reattached cases, there was an average bubble reabsorption time of 92 days, whereas those with failed cases had an average reabsorption time of 62.7 days.81 Surgeons generally seek nearly total fill with a long-acting gas bubble. Nonexpansile or only slightly expansile concentrations of the gas are used to avoid extremely high pressures that could cause central retinal artery occlusion. It is important to monitor these patients postoperatively so that some gas can be removed to relieve elevated pressure if necessary. Although applanation tonometry is the most accurate means of following intraocular pressure, visual acuity monitoring for the loss of light perception by the nurse or by the patient can be used as the earliest sign of excessively high pressure. In measuring intraocular pressure in patients with a gas bubble, large displacement tonometry such as Schiotz tonometry gives falsely low values. A correction factor based on the size of the gas bubble is necessary. Applanation technique such as Goldmann tonometry or the use of electronic penlike devices is much more accurate. With large bubbles used in PVR surgery, face-down positioning is important to prevent pupillary block glaucoma and to minimize the risk of peripheral anterior synechiae formation. The higher surface tension and higher buoyant forces of the intraocular gas bubbles relative to silicone oil result in better internal tamponade with a gas bubble. The volume of the gas bubble is constantly decreasing, however, whereas a silicone oil bubble remains at constant volume. The gas bubble volume can be easily supplemented using an outpatient fluid–gas exchange if the bubble is too small or disappearing too rapidly. The most frequent complication of PVR surgery is recurrent PVR and retinal re-detachment. In the cases that remain attached, macular pucker is the most frequent complication (seen in 30% of patients).82 These complications are not due to a toxic effect of the gas but seem to be due to the displacement of fluid, which may concentrate proliferative factors near the retinal surface.
The use of gas has been generalized to treatment of any retinal break at the end of vitrectomy.83 Although retinal breaks do not routinely require gas tamponade in a simple scleral buckling procedure, the situation is different after vitrectomy. The replacement of slightly viscous vitreous fluid and more viscous subretinal fluid with low-viscosity BSS permits transitional and more turbulent flows with ocular motion. These flows create detachment forces around retinal breaks. Furthermore, these flows persist longer because of prolonged damping times in lower viscosity fluids. If small or even large amounts of subretinal fluid remain at the end of the vitrectomy, the retina can be flattened postoperatively by the gas bubble with appropriate prone or lateral positioning.
To illustrate the advantage of stabilization of retinal motion by leaving the viscous subretinal fluid in place until the end of vitrectomy but before fluid–gas exchange, visualize two jars with a tissue suspended in fluid in each. One jar has water in it, while the other jar has honey in it. Twist both jars rapidly a few times, then wait for the fluid motion to stop. Obviously, the jar with honey in it will have the fluid motion come to rest much more quickly. The decay times are proportioned to the reciprocal of the square root of the Ekman number.84 The Ekman number is a measure of comparison of viscous forces to Coriolis forces in such a system. In a system with relatively fixed rotational and physical properties such as the eye, the damping time will be proportional to the reciprocal of the square root of the viscosity of the subretinal fluid. A viscous subretinal fluid may have a viscosity of around 100 cP whereas BSS has a viscosity of 0.7 cP. Thus the damping time would be 12 times shorter and stabilization of the retina would be 12 times greater with the subretinal fluid in place.
Pneumatic retinopexy is frequently used in retinal detachments in which tears occur in the superior 8 clock hours. There are reports of reattachments with pneumatic retinopexy in patients with inferior tears and also in those with tears at multiple clock hours superiorly.85 These techniques require either face-down positioning for the inferior tears or sequential postural positions for two or more tears at significantly different clock hours. When pneumatic retinopexy is used for one or more tears all located within 1 clock hour in the upper 8 clock hours of the eye, the primary retinal reattachment rate is similar to that of scleral buckling.86 In failed pneumatic retinopexy cases, reoperation with scleral buckling or vitrectomy gives a final success rate comparable to the final success rate for patients initially treated with scleral buckling. In the subset of patients with recent macular detachment and a subtotal detachment, visual acuity results are superior to those with scleral buckling. This would suggest that pneumatic retinopexy may be the procedure of choice for this subset of patients. Pneumatic retinopexy is more successful at reattaching the retina on a single procedure basis with phakic patients than with aphakic patients.87 The gas is injected with a small-gauge needle through the pars plana. Production of a single bubble is desirable because multiple small bubbles may go through a larger retinal tear. If multiple small bubbles are obtained, the bubbles may coalesce by flexing a cotton-tipped applicator and then releasing its tip to firmly strike the pars plana of the eye. If this technique does not work, the patient is usually positioned with the bubbles in the direction opposite from the tear overnight so the bubbles will coalesce. This is especially important if the break is large enough to allow passage of bubbles. Cryopexy can be performed before injection of the gas bubble or subsequent to flattening the retina. If laser treatment is used, it requires flattening of the retinal break and is usually performed on the second or third postoperative day. Relatively small amounts of gas, on the order of 0.3 to 0.6 mL. are injected. In patients suspected of having glaucoma, either the procedure should be avoided or intraocular pressure and volume should be lowered enough to accommodate the entire gas bubble before injection. In emergency situations, anterior paracentesis can be performed in phakic or capsule-intact pseudophakic patients. Paracentesis of the anterior chamber through the pars plana can be performed on aphakic patients. When the gas bubble is adequately coalesced, usually shortly after the time of injection, the patient is positioned for 16 hours a day such that the bubble lies directly beneath the retinal break. This position tamponades the tear, thus allowing spontaneous reabsorption of the fluid by the choroid. A steamroller maneuver, in which the patient is placed face down and then slowly lifts his or her head, can be used to propel a substantial amount of subretinal fluid from under the retina in patients with larger breaks. This procedure can speed up retinal flattening, but it is not absolutely necessary for retinal reattachment. Delayed reabsorption of subretinal fluid has been noted in patients with subretinal precipitates and in cases with heavy cryotherapy.88 Sulfur hexafluoride gas is the most frequently used gas, but perfluoropropane may be used if a longer tamponade is desired. Air, perfluoroethane, and octofluorocyclobutane have also been successfully used in pneumatic retinopexy.
Intraocular gas injections, which are essentially modified pneumatic retinopexy procedures, are frequently used to rescue recurrent detachments from a new or previously undetected retinal break in scleral buckling and postvitrectomy cases. The gas is injected through the pars plana. The head is positioned so as to tamponade the tear. Either indirect or slitlamp laser photocoagulation is preferred because cryopexy is often impossible due to the silicone buckle. Because vitrectomy and a scleral buckle have already been performed, intraocular air may be sufficient in these cases. If recurrent PVR is suspected, longer-acting gases are preferred.
A postvitrectomy fluid–gas exchange is frequently performed to remove persistent vitreous hemorrhage, especially in diabetic retinopathy. Because the vitreous fluid is now modified aqueous, the exchange can be performed at the bedside or in the office. A 10-mL syringe filled with 5 mL of sterile air or nonexpansile gas is used with a 25-gauge needle. The needle entry is through the limbus in aphakic patients or through the pars plana in phakic or pseudophakic patients (Fig. 54-5). The patient is positioned so that needle entry is from below. Fluid is aspirated, and gas is injected alternately until most of the hemorrhagic liquid is replaced with gas. This procedure allows supplemental laser photocoagulation and temporary removal of proliferative factors in the hemorrhagic vitreous fluid.
Macular holes in undetached retina are treated with vitrectomy in combination with membrane dissection and fluid–gas exchange.89 Subsequent macular hole surgery experience demonstrates that successful surgery requires at least removal of the posterior hyaloid face over the disc and macula and tamponade with a large gas (usually 16% perfluoropropane) or silicone oil bubble. Epiretinal membranes are usually removed if present. Internal limiting membrane removal is controversial. There is a suggestion of higher closure rates with indocyanine green–assisted internal limiting membrane (ILM) peeling,90 but a question whether this is traumatic or toxic to the retinal pigment epithelium (RPE) and retina remains.91 However, more recent reports using preoperative evaluation of the photoreceptor ultrastructure with optical coherence tomography (OCT) suggests that there is no significant additional trauma to ILM peeling.92 ILM peeling is usually performed in long-standing macular holes or reoperations. Various adjuvants such as transforming growth factor β, plasma, serum, and platelet concentrate have not been shown to confer a significant advantage.
Treatment of macular detachment secondary to optic nerve pits has been performed with intraocular gases. A pneumatic retinopexy treatment with prone positioning to flatten the retina in the papillomacular bundle region is performed initially. A barricade of laser is then placed across the papillomacular bundle region separating the optic nerve pit from the macula. If this is not successful, vitrectomy and a larger gas bubble with face-down positioning and repeat laser photocoagulation can be done. This procedure is warranted since a natural history study showed a very high percentage of visual loss with chronic macular detachment caused by optic pits.
COMPLICATIONS OF INTRAOCULAR GASES
The complications of corneal opacity and cataract formation resulting from drying by gas bubbles have been noted already. Face-down or lateral positioning is necessary to prevent continuous contact of the gas bubble with the cornea and lens and to minimize the risk of corneal opacity and cataract formation.
Glaucoma can develop from association with intraocular gas bubbles. Overfilling of the eye with expansile gas and consequent central retinal artery occlusion have led to permanent blindness.93 Medium-size fills and inadequate attention to prone positioning can cause peripheral anterior synechiae with total angle closure. Sharp increases in intraocular pressure can occur if the patient remains supine, because fluid from the ciliary body continues to fill the posterior segment while the air bubble blocks fluid egress through the trabecular meshwork. Changes in atmospheric pressure, such as air travel or driving rapidly up a steep mountain, can lead to painful pressure increases and loss of vision. To avoid loss of vision in patients with a gas tamponade due to nitrous oxide anesthesia during an unanticipated emergency, a gas identification bracelet should be issued at the time of their vitreoretinal procedure.
Indirect and slitlamp laser treatment can cause undesirable burns from reflections of internal fluid–air and air–fluid surfaces. To reduce this risk, one should avoid treatment through a gas–fluid or fluid–gas interface whenever possible.94 When treatment through such an interface is unavoidable, it should be done as perpendicular to the interface as possible, because the intensity of a reflected beam increases as the angle of incidence decreases. A divergent beam should be used. The location and the intensity of internally reflected beams should be evaluated with the aiming beam before photocoagulating the area of interest. Polarization of the laser beam could potentially reduce the problem of internal laser reflectance. Changes in beam intensity caused by reflectance and relative magnification encountered when switching from treating through a gas bubble to treating around it can lead to overintense burns and result in retinal and choroidal hemorrhages.
Some complications are particularly important to pneumatic retinopexy. Endophthalmitis has been encountered. Undiluted povidone-iodine (Betadine) solution was not used in most of these cases. The use of povidone-iodine is exceptionally important in pneumatic retinopexy or any transconjunctival injection into the vitreous because it is bactericidal. Topical antibiotics are not adequate because they require a long time to act and may be merely bacteriostatic rather than bactericidal. The conjunctiva should be dry before injection through the site to avoid placing potentially contaminated and iodine-containing fluid into the vitreous cavity.
Endophthalmitis has been reported with the use of gases in vitrectomy surgery, but it is not clearly related to the use of the gas. Gas for intraocular injection is aspirated through a 0.22-μm filter for sterilization. Many surgeons use two such filters in series to reduce risk of contamination by failure of one filter membrane. If the injecting needle used during pneumatic retinopexy is not first inserted deep into the vitreous before pulling it back to approximately 2 mm, gas can be trapped in the anterior hyaloid. This produces a donut- or sausage-appearing gas pocket anteriorly (Fig. 54-6). The gas may break free into the vitreous after 1 or 2 days. Many surgeons prefer to aspirate the gas immediately and reinject it deeper into the vitreous. If multiple small bubbles are formed at injection and not eliminated before positioning them beneath the retinal tear, subretinal gas may be found. New tears occur in 7% to 23% of patients treated with pneumatic retinopexy. New tears are also found in approximately 13% of patients treated with scleral buckling. Iris-supported intraocular lenses may flatten against the cornea after posterior fluid–gas exchange. Several remedies have been proposed for this problem, including injection of hyaluronic acid into the anterior chamber, injection of air into the anterior chamber, and placement of anterior chamber sutures to prevent the implant from touching the corneal endothelium.95 Fortunately, the implantation of this kind of lens is now rare. The rigid angle-supported anterior chamber lens and the posterior chamber intraocular lens rarely touch the cornea with fluid–gas exchange of the posterior segment.
AVAILABILITY AND FDA STATUS OF INTRAOCULAR GASES
Perfluoropropane and SF6 have been approved by the FDA for commercial distribution. Two surveys have shown that the use of SF6 and perfluoropropane have long been the standard of care in the United States.96 The longer-acting gases were preferred over air for pneumatic retinopexy, treatment of posterior tears, and treatment of PVR. There was a slight preference of perfluoropropane for vitrectomy and sulfur hexafluoride for pneumatic retinopexy.
SILICONE OIL AS A VITREOUS SUBSTITUTE
Silicone oils, first available in 1945, were developed as a replacement for hydrocarbon lubricants and rubbers. They were used for breast augmentation in the mid 1950s. Silicone oils were proposed as a vitreous substitute in 1958 but were first used for ocular surgery by Cibis et al97 and Armaly98 in 1962. Although Cibis et al97 were successful at reattaching PVR detachments that were otherwise inoperable at the time; their work predated the development of vitrectomy.
Because of early clinical and laboratory reports of severe silicone oil retinopathy and because of the subsequent development and success of vitrectomy surgery for PVR, silicone oil was essentially abandoned in the United States in the late 1960s and 1970s.99 Scott100 continued to use silicone oil with a viscodissection technique in Great Britain. He performed dissection of vitreous membranes with the indirect ophthalmoscope using needles and early vitrectomy scissors. Haut et al,101 in France, were the first to use silicone oil as a modern tamponade after vitrectomy. In Holland, Zivojnovic et al102 aggressively developed the full potential of silicone oil as an adjunct to vitrectomy surgery. Laganowski103 made a major contribution to the use of silicone oil with vitrectomy by noting that a 6 o’clock peripheral iridectomy substantially reduced the incidence of silicone keratopathy in aphakic patients. In 1992, a multicenter randomized trial showed that silicone oil was superior to SF6 and equal to perfluoropropane in repairing PVR detachments in conjunction with vitrectomy.104
MODE OF ACTION AND PHYSICAL PROPERTIES OF SILICONE OILS
The chemistry of silicone oil begins when silicone dioxide (sand) is reacted with carbon at a high temperature, resulting in silicium.105 Silicium is reacted with methyl chloride, whose product is then reacted with water. The result is dimethylsilanediol, which polymerizes into long chains of silicium oxygen molecules with two methyl groups on each silicium. The polymer chains continue to grow in length until a trimethyl group is placed at each end of the chain. The greater the length of the polymer, the greater the viscosity of the resulting silicone oil. During the synthesis of silicone oil, mixtures of different chemical substances can result. Therefore, the chemical composition of a particular silicone oil depends on the manufacturing process.
The most commonly used silicone oil clinically is methyl-3,3,3 polydimethylsiloxane. This is the familiar silicone oil that is lighter than water. The methyl groups, however, can be substituted with trifluoropropylmethyl or methylphenyl side chains, which result in heavier than water silicone oil.
The silicone oil chains form a helix with approximately six silicium units per turn. A pure 1,000-centistoke oil will have a helix of 63 turns and a 5,000-centistoke oil a helix of approximately 100 turns (Fig. 54-7).106 Interdigitation of the helix accounts for part of the viscosity, as does the increasing molecular weight of the larger molecules. Temperature dependence of the viscosity of silicone oil is shown by the fact that 1,000-centistoke dimethylsiloxane at room temperature has a viscosity of 800 centistokes at body temperature.
Because aqueous and silicone oils are immiscible, buoyancy occurs. The density of aqueous humor is 1.01 g/mL. The density of regular silicone oil at body temperature is 0.96 g/mL, and the density of the trifluoropropylmethylsiloxane at body temperature is 1.29 g/mL. The lighter silicone oil floats on the water, whereas the heavier silicone oil sink beneath the aqueous phase. The buoyant force of a regular silicone oil bubble with a volume of 5 mL is approximately 0.25 g. The downward force of the fluorinated silicone oil can be as much as 1.3 g. This downward force is distributed over the surface of the bubble and is greatest in the directly vertical axis. It tapers off to zero on the retinal surface parallel to the vertical axis. For regular silicone oil, the most important implication of buoyancy is that it directs the tamponade of the immiscible silicone oil upward. With the heavier-than-water silicone oils, the negative buoyancy is sufficiently great that it becomes a valuable intraoperative tool in expressing subretinal fluid from beneath the retina through peripheral tears. The heavier oils are also valuable in defining residual membranes on the retina by putting them on the stretch.
Surface tension is the most important means by which silicone oil assists in tamponading retinal tears for retinal reattachment. Surface tension between air and liquid was discussed earlier. The interface between silicone oil and water is somewhat more complex. The short-range forces at the surface that give rise to surface tension are diminished between the water/oil interface relative to the water/air interface. This is because there is some attractiveness between the water and oil molecules that diminishes the dispersive forces and, therefore, the surface tension (Fig. 54-8). Strong polar forces give a further boost to surface tension at the water/hydrocarbon interface. Strong polar forces play a similar role in the silicone oil/water interface. Although in a successful retinal reattachment no fluid is seen in the area of successful tamponade, the retina itself and the choroid underlying the tamponaded retinal break may be thought of as being an aqueous phase material.
A rough theoretical calculation gives the surface tension between pure water and regular silicone oils as approximately 50 ergs/cm2,107 An actual measurement of pure water and silicone oil was 40 ergs/cm2. When physiologic saline was used, surface tension was found to be 33 ergs/cm2. If hyaluronic acid solution is used during the surgery and remains in the aqueous phase, surface tension may be reduced to 25 ergs/cm2.
The surface tension may be further decreased by surfactants or surface-active agents. Surfactants coat the interface between the oil and the aqueous phase vitreous fluid since they are partially soluble in both phases. This makes the transition between the two immiscible liquids less abrupt.108 The most important van der Waals forces at the interface are of very short range (on the order of one molecular layer). Thus, a surfactant that is one molecular layer thick can substantially decrease the dispersive force at the surface of the liquids. Because the dispersive force is decreased, there is less crowding at the interface and less surface tension. In ocular surgery, proteins and phospholipids act as surface-active agents intraocularly. Proteins, however, are least effective at physiologic pH. Therefore, phospholipids are probably the best candidates for natural intraocular surfactant.109
The surface tension of silicone oil can be reduced to 12 to 14 ergs/cm2 by the absorption of lipoprotein. When 1,000-centistoke silicone oil or fluorosilicone oil was placed against liquified bovine vitreous, it had an interfacial tension of 14 ergs/cm2.110 After silicone oil has been in the eye, its surface tension may become so low that it can easily slip through a retinal break.
The concept of first flattening the retina with air, which has a much higher surface tension than silicone oil, is important. If the retina cannot be flattened by an air–fluid exchange because of residual traction, silicone oil cannot do so because its surface tension against the retina and choroid is less than that of air. According to Boyle’s law, the pressure differential across the silicone aqueous interface is proportional to two times the surface tension divided by the radius of the bubble. As the pressure differential rises across the silicone oil/subretinal fluid interface in the plane of a break, a small hemispheric bubble is formed on the subretinal fluid side.111 The maximum pressure difference that the surface of the bubble can sustain is reached when the radius of curvature of the bubble is equal to the radius of the break (Fig. 54-9). Any further increase in pressure forces silicone oil under the retina. As a surgical rule, oil rarely goes under the retina if the retina is closer to the choroid than the diameter of a 20-gauge cannula.
A further problem with decreasing surface tension of silicone oils over a period of time is the development of emulsification.112 Spontaneous emulsification without physical motion occurs as the surface tension approaches zero. As a practical rule, emulsification occurs with surface tension in the range of 6 to 15 ergs/cm2. Surfactants alone probably do not lead to spontaneous emulsification of clinical silicone oils. Ocular motion can lead to emulsification, especially as surface tension decreases. Again, viscosity is important. Lower-viscosity silicone oils emulsify more rapidly than higher-viscosity silicone oils.113 Ocular fluid motion with instability at the interface leads to formation and separation of tiny droplets. Droplet formation decreases with increasing viscosity, because increasing viscosity dampens unstable motion at the interface. This notion was discussed under the topic of the Reynolds number in the section on viscous vitreous substitutes. Thus, a higher-viscosity silicone oil emulsifies less easily than a lower-viscosity oil even if the surface tension is the same.
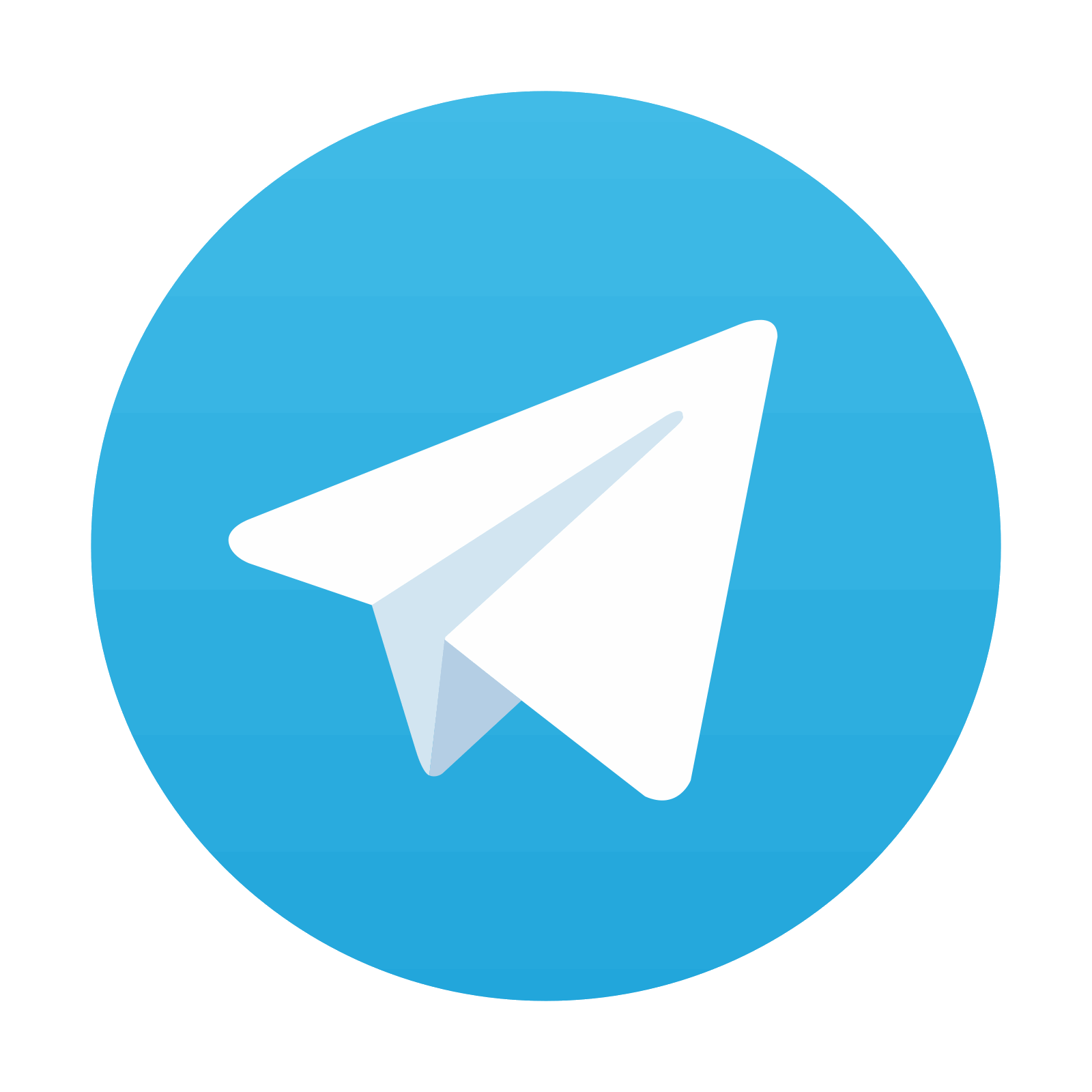
Stay updated, free articles. Join our Telegram channel
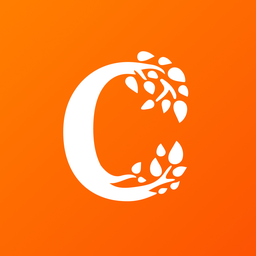
Full access? Get Clinical Tree
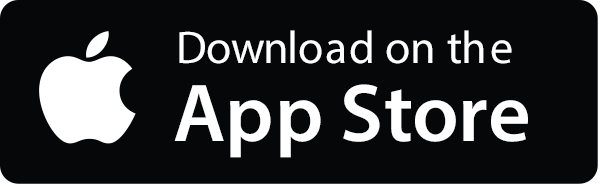
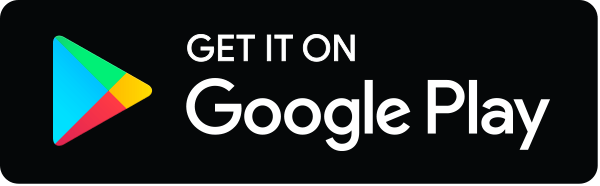