Vitreous Humor
George A. Williams
Alan J. Ruby
Mark S. Blumenkranz
VITREOUS STRUCTURE
General Features
The vitreous of the normal human eye weighs approximately 4 g and occupies a volume of almost 4 mL. The precise weight and volume vary with the age and the size of the eye. The vitreous body is spherical with a depression in the anterior surface, the patellar fossa, corresponding to the posterior surface of the crystalline lens. Larsen1 ultrasonically measured the axial length of the vitreous body in 926 children. He noted that the mean value in newborns was 10.48 mm in boys and 10.22 mm in girls. A sex difference of 0.23 to 0.38 mm greater mean length was noted in males at birth and persisted throughout the growth period. By the age of 13 years, when the axial growth of the vitreous body is essentially completed, the average length was 16.09 mm in males and 15.59 mm in females. Larsen further noted that the relationship between vitreous length and refraction was already established by 1 year of age.
The vitreous body can be divided into two zones or regions. The more peripheral zone, the cortical vitreous, encases the medullary vitreous. The cortical vitreous consists of a relatively more condensed, fibrillar vitreous. Although the cortical vitreous represents only 2% of the total vitreous volume, it is the metabolic center of the vitreous body, because it contains the hyalocytes.2,3 Another connective tissue cell, the fibrocyte, is also found in the cortical vitreous. Most of the vitreous body, the medullary vitreous, is essentially a cell-free mixture of collagens and hyaluronan (HA) existing either in a gel or a liquid state depending on the age, refraction, and condition of the eye.
The vitreous body interfaces with several ocular structures through the vitreous cortex (Fig. 11-1). The vitreous cortex extends anteriorly from the vitreous base to form the anterior vitreous cortex and posteriorly to form the posterior vitreous cortex. Recently, the clinical importance of vitreous cortex has become increasingly apparent. The vitreous cortex has been implicated as a primary factor in various vitreoretinal disorders, including retinal breaks, proliferative vitreoretinopathy (PVR), anterior hyaloidal fibrovascular proliferation, macular holes, epiretinal membranes, diabetic retinopathy and age-related macular degeneration.
Anterior Vitreous Cortex
The anterior vitreous cortex or anterior hyaloid is the anterior surface layer or condensation of the vitreous body. There is no specialized membrane that constitutes the anterior vitreous cortex, but rather, there is a greater density of collagen fibrils. Microscopically, the framework of collagen fibrils that run throughout the vitreous body ends in an interwoven network to form the anterior vitreous cortex. The superficial fibrils tend to run parallel to the surface in this region. The smooth surface and membrane-like appearance are owing to the lamellar distribution of the cortical fibers and the associated highly polymerized mucoproteins.4,5 Anatomically, the anterior hyaloid forms the posterior limits of the posterior chamber. This portion of the vitreous cortex functions in the physiologic communication between the vitreous cavity and the aqueous humor. The anterior surface of the vitreous body separates from the pars plana approximately 1.5 mm anterior to the ora serrata. It extends medially to contact the lens posterior to the lens equator. Thus, the anterior hyaloid is in contact with the ciliary processes and the lens zonules, as well as the posterior lens capsule. The vitreous attaches to the lens capsule in a ringlike manner, forming the hyaloideocapsular ligament of Wieger. This ligament is believed by some to be synonymous with the attachment ring of the posterior zonular fibers.4 The circular area of attachment measures approximately 9 mm in diameter and is especially firm in younger persons or after intraocular inflammation.5 In this circular area the anterior hyaloid is thickened.
Central to the attachment of the Wieger ligament (also known as the Egger line), the vitreous lens attachment is less pronounced and appears to be owing to surface tension. This central area contains a potential space within the 9-mm ring known as the Berger space, or the patellar fossa. The anterior hyaloid then turns posteriorly to form the anterior portion of the Cloquet canal in the midportion of the Berger space. The Cloquet canal represents the remnants of the primary vitreous and can sometimes be seen with the slitlamp. It arises from the optic disc in a funnel-shaped manner, in the area of Martegiani, and extends forward to the posterior lens surface. The canal is 1 to 2 mm wide and has a downturn in the central vitreous cavity. The area of contact with the posterior lens capsule can at times be identified by a tag of embryonic tissue, known as a Mittendorf dot, located slightly nasal to the posterior pole of the lens. Similarly, a remnant of the posterior primary vitreous can occasionally be identified on the optic disc. This remnant, representing the embryonic point of exit of the hyaloid vascular system from the optic nerve head, is known as the Bergmeister papilla. The walls of the Cloquet canal are formed by a vitreous condensation rather than a true membrane (Fig. 11-1).
Posterior Vitreous Cortex
The mechanical relationship between the vitreous and the retina is mediated by the posterior vitreous cortex, which is also called the posterior hyaloid. The posterior vitreous cortex consists of relatively densely packed type II collagen fibrils arranged tangentially to the retina. The retinal basal lamina is the basement membrane of the Müller cells that compose the internal limiting membrane (ILM) of the retina.6,7 Ultrastructurally, the ILM consists of three layers.8 Adjacent to the end feet of the Müller cells is the lamina rara interna. The lamina rara externa is contiguous with the vitreous cortex. In between these layers is the lamina densa. Collagen fibers of cortical vitreous are tangential to the lamina rara externa. The ILM is composed of primarily type IV collagen but also contains fibronectin, laminin, and type I collagen.9 The morphology of the ILM varies topographically in the retina. The ILM, and in particular the lamina densa, is thin in the retinal periphery and becomes increasingly thicker and irregular in the posterior retina.6,8 The ILM thickens from 50 nm at the vitreous base to 300 nm at the equator to 1,900 nm posteriorly. In the foveal region the ILM thins to 10 to 20 nm.10
The nature of the adhesion between the vitreous cortex and the ILM is enigmatic. This adhesion is strongest at the optic nerve, the macula, the vitreous base, and retinal vessels. Foos6 demonstrated attachment plaques between Müller cell cytoplasm and the ILM in the basal and equatorial retina (Fig. 11-2). Vitreous traction mediated by vitreous fibrils may contribute to these adhesions. These attachment plaques are not present posterior to the equator except where the ILM is thinned in the fovea. This anatomic variation in the fovea region may play a role in the pathogenesis of some of the vitreomacular disorders.
The ILM also thins over major blood vessels. Vitreous strands extend through pores in the ILM to surround the vessels11 and have been termed vitreoretinovascular bands.12 These bands may explain the strong adhesion between the vitreous and retinal vessels.
Despite the presence of these anatomic interfaces between the vitreous cortex and ILM, the adhesion of these structures is still not completely understood. Biochemical investigations suggest that vitreoretinal adhesion involve molecular adhesion mediated by glycoproteins such as fibronectin, laminin, and other glycoconjugates. The ILM consists of type IV collagen, laminin, fibronectin, type I collagen, and as yet uncharacterized glycoproteins. It is believed these substances mediate vitreoretinal adhesion.13
The ILM undergoes age-related changes that are anatomic, biochemical, and functional. Anatomically, the ILM thickens with age. Biochemically, the distribution of fibronectin and laminin changes with age. In young eyes, antifibronectin antibody binding has a homogeneous distribution. In older eyes, the posterior ILM shows a segmented bilaminar distribution of both antifibronectin and antilaminin antibodies.14 An age-dependent distribution of other ILM glycoconjugates has also been demonstrated. Variations in the distribution of these and other glycoproteins may play a role in conditions that are characterized by alterations in vitreoretinal adhesion.15
VITREOUS BASE
The vitreous base is a three-dimensional zone centered on the ora serrata where the vitreous is the most adherent to the retina and pars plana epithelium. It extends approximately 1.5 mm anteriorly to the ora serrata. Nasally, it extends approximately 3.0 mm posterior to the ora and 2.0 mm posterior to the ora temporally. The functional base of the vitreous extends several millimeters into the vitreous body in this region. As the eye ages, the firm attachment of the vitreous base extends further posteriorly. This may lead to localized areas of enhanced vitreoretinal traction and result in retinal tears.
In the vitreous base, collagen fibers are relatively coarse, are numerous, and insert perpendicularly to the retina and pars plana. These fibers consist of collagen fibrils with diameters of 10.8 to 12.4 nm.16 The microscopic detail of the vitreous fibers’ attachment to the vitreous base varies from anterior to posterior. Anterior to the ora the fibers are less dense than posterior to the ora. Electron microscopic studies demonstrate that the vitreous fibers have complex interdigitations with the reticular fibrillar materials of the basement membrane of the nonpigmented ciliary epithelium but do not pass between the cells.17 The anteriormost fibers splay out anteriorly to form the anterior loop of the vitreous base, which is important in the pathogenesis of anterior PVR.18 Posterior to the ora, bundles of vitreous fibrils attach to the ILM. Cords of vitreous collagen insert into gaps between the neuroglia. Gloor and Daicker19 likened this arrangement to Velcro and suggested that this may explain the strong vitreoretinal adhesion of the vitreous base. Cellular elements are also present in the vitreous base. Fibroblast-like cells are present anterior to the ora, and macrophage-like cells are posterior to the ora.17 These cells may play a role in the hypocellular gel contraction that characterizes anterior PVR.
VITREOUS CELLS
The vitreous normally contains relatively few cells compared with other connective tissues. Most of these are found within the thin layer of cortical vitreous. These few cells account for the metabolic activity of the vitreous body, which is among the lowest in the body. The cells are variously called vitreous cells, vitreous cortex cells, vitreocytes, or, most commonly, hyalocytes. In the physiologic state, the vitreous body appears to have a mixed population of cells. Morphologically, most are hyalocytes, whereas approximately 10% are similar to fibroblasts.
Hyalocytes are found throughout the vitreous cortex, but they appear to be concentrated in the basal region.3 These cells are more numerous in the fetal vitreous than in older eyes, and their exact origin and function are open to question. Some investigators believe hyalocytes are remnants of the primary vitreous.20 Based on developmental studies in animals and humans, hyalocytes arise from either monocytes originating from mesenchymal cells in the optic cup or embryonic fissure or from blood elements in the vasa hyaloidea propria. These cells migrate through the vitreous cavity and accumulate in the cortical vitreous as hyalocytes by the fifth month of gestation. Following birth, there is no further migration of cells to the vitreous cortex and existing hyalocytes do not normally proliferate. As a result, ocular growth results in increasing vitreous cortex surface area with a corresponding decrease in hyalocyte density. Gloor21 contests this hypothesis and believes hyalocytes are continuously derived from blood monocytes through life. The hyalocytes remain in the vitreous cortex for about a week and are then replaced by blood-borne monocytes to maintain a constant cell population.
The hyalocytes appear as flattened, 10 to 15 μm in diameter, spindle-shaped cells when found over the retina, whereas in the region of the vitreous base, the cells are larger, rounder, and at times star-shaped with prominent nuclei.21,22 The hyalocytes are active metabolically with numerous lysosomal granules, phagosomes, and Golgi complexes. Hyalocytes synthesize vitreous glycosaminoglycans (GAGs) including HA and hexosamine.23 The highest density of hyalocytes is in the region of the vitreous base followed by the posterior pole, with the lowest density at the equator.
In addition to the hyalocytes and fibroblasts, there are cells found in the vitreous that appear to be in varying stages of degeneration.24 These cells are believed to represent the involutional remnants of the original cell population of the embryonic vitreous and are seen more often in young eyes. By electron microscopy, in the region of the ora serrata, cells are seen that appear to be damaged retinal cells that have been sloughed into the vitreous base.17
There is a mechanism for the elimination of foreign and degenerated substances from the vitreous that is found partly in the region of the optic disc. When India ink is injected into the anterior portion of the vitreous in a rabbit eye and the enucleated eye examined at varying intervals, it is noted the ink particles are eliminated from the vitreous by way of the optic nerve.25 Later the particles are removed by phagocytic cells that appear to enter the vitreous body from the region of the optic nerve and ciliary body. Surgical treatment may also affect the cellular composition of the vitreous. Cryopexy induces a macrophage influx into the vitreous cavity.26 This may play a role in the clearing of vitreous opacities.
VITREOUS MOLECULAR STRUCTURE
In this section we present a brief overview of the macromolecular structure of the vitreous. For a more complete discussion of this complex area, the reader should consult the excellent works of Sebag,27 Balazs,28 and Bishop.29
The major macromolecular components of the vitreous are HA and collagens. HA is a GAG consisting of repeating disaccharide units of glucuronic acid and N-acetyl glucosamine. It is arranged in a linear helix that undergoes conformational changes into both a compressed and extended conformation. The molecular weight of the sodium salt of HA ranges from 3 to 4.5 × 106. In vivo, HA is highly hydrated with a large specific volume of 2,000 to 3,000 mL/g. This results in the formation of a highly entangled open coil. HA is produced by the hyalocytes that possess the necessary enzymes for HA synthesis. HA concentration is highest in the regions of greater hyalocyte density. Therefore, it is highest in the cortical gel.
Vitreous collagen consists of nonbranching fibrils 7 to 28 nm in diameter. The collagen molecules forming these fibrils are covalently linked. Transmission electron microscopy reveals that the fibrils have uniform banding density and are associated with a filament consisting of a succession of linked 20-nm beads with a spacing ranging from 35 to 85 nm. The vitreous base has the highest density of collagen fibrils, followed by the posterior and then anterior vitreous cortex.30
The fibrils consist of a mixture of collagen types distributed along each fibril. Semiquantitative analysis of bovine vitreous reveals collagen types II, V, and IX distributed in a ratio of 69:24:7, respectively.30 Although most of the collagen is type II, types IX and V are believed to be important in promoting stability of the vitreous gel. Type IX collagen probably promotes cohesion between the fibrils and HA. Type V collagen coats the type II collagen to produce a continuous filament structure in the fibril. The assembly of these collagens results in a fibril of enhanced stability and strength.
There may be some topographic variation in vitreous fibril composition. Transmission electron microscopy of the vitreous base shows fibrils consistent with type VI collagen.30 It is the interaction between the collagen fibrils and HA that provides the vitreous gel with its unique mechanical and optical properties. The large domains of HA stabilize and spread apart the collagen fibrils, thus minimizing light scattering. The viscoelastic properties of vitreous are the result of complex molecular interactions between the collagen fibrils and HA (Fig. 11-3).
Scott31 has suggested that there is some order to the association of the vitreous GAGs and collagens. He proposed a three-component complex that forms an infinite meshwork contributing to the semirigidity of the vitreous. The spaces between collagen fibrils are filled by HA and other GAGs such as chondroitin 6-sulfate. The GAGs link together as aggregates via hydrophobic and hydrogen bonding, resulting in a three-dimensional honeycomb structure. This ordered aggregation of GAGs is fragile and may have clinical implications. HA fragments may destabilize the aggregation of GAGs.32 Therefore, a small amount of HA breakdown may have a marked effect on the integrity of the whole gel. HA is sensitive to damage by free radical mechanisms. Irradiation of the vitreous with 300-nm light or with white light in the presence of photosensitizers results in partial depolymerization of HA. Exposure to neodymium:yttrium-aluminum-garnet (Nd:YAG) laser energy has a similar effect. These photodynamic events generate hydroxyl radicals that are responsible for the HA degradation. Depolymerization alters the hydration of HA, resulting in liquefaction. This mechanism may explain the vitreous liquefaction seen with age, inflammation, or after cataract extraction.
The vitreous contains a group of enzymes known as matrix metalloproteinases (MMPs), which are zinc-dependent enzymes that mediate the structure and function of the extracellular matrix (ECM). Because most of the vitreous is ECM, it is not surprising that MMPs are present and that MMPs have been implicated in various vitreoretinal disorders. The enzymatic activity of MMPs is regulated by tissue inhibitors of metalloproteinases (TIMPs). The functional proteolytic activity of MMPs in the vitreous is thus dependent on the relative concentrations of regulatory TIMPs and active MMPs. There are at least 18 MMPs and 4 TIMPs that have been reported. The MMPs have varying substrate specificity and patterns and location of expression. In the vitreous, MMP-1, MMP-2, MMP-3, and MMP-9 are present. TIMP-1, TIMP-2, and TIMP-3 have also been reported in the vitreous. The source of vitreous MMPs and TIMPs is not known, but hyalocytes may be responsible.33
MMPs and TIMPs play both a physiologic and a pathologic role in the vitreous. These enzymes are probably responsible for the proteolytic state that clears the vitreous of potential opacification and maintains optical clarity. In disease states, MMPs have been implicated in PVR, retinal neovascularization, retinopathy of prematurity, and proliferative diabetic retinopathy.33,34
PATHOPHYSIOLOGIC MECHANISMS IN VITREOUS DISEASES
There are two distinct mechanisms by which alterations in vitreous morphology lead to clinical disease syndromes: mechanical interfacial separation of the vitreous from the retina, and cell-mediated contraction of the cortical vitreous gel.
MECHANICAL INTERFACIAL SEPARATION OF THE VITREOUS FROM THE RETINA
Mechanical separation of the vitreous from the retina occurs when the vitreous separates from the ILM of the retina. This separation may be relatively complete, as in posterior vitreous detachment (PVD), or may be limited, as in macular hole formation. PVD occurs when mechanical stress forces acting on the vitreous body overcome the naturally occurring adhesion of the vitreoretinal interface.
PVDs are usually spontaneous events that are age related, but they may also be precipitated by trauma. They occur earlier in association with vitreous inflammation, vitreous hemorrhage, myopia, and diabetes. As the vitreous ages, there is an increase in the water content and subsequent liquefaction of the vitreous, a process that normally begins by the age of 5 years. At about 40 years, the volume of the vitreous gel begins to decrease in parallel with the increase in liquid vitreous until approximately half the vitreous space is occupied by liquid vitreous (Fig. 11-4). This process of liquefaction is called synchysis. Synchysis usually begins in the midvitreous and is recognized with biomicroscopy as optically empty spaces without the membranous appearance of the normal vitreous. This is because liquid vitreous does not contain collagen fibrils.
![]() Figure 11.4. Change of the liquid and gel vitreous volume during aging and development of the human eye. |
Once vitreous liquefaction occurs, fluid currents develop within the vitreous. These currents, stemming from the rotational and gravitational forces transmitted to the vitreous body, produce stresses at the vitreoretinal interface that lead to separation at points of weakest attachment. Dark-field slit microscopy studies on human autopsy specimens indicate that persons 21 years or younger demonstrate firm adherence between the ILM and the vitreous cortex in the posterior pole. Older persons demonstrate relatively weak adhesive forces in comparable areas.15
After liquid vitreous has formed, vitreous body collapse or syneresis can occur. This event is precipitated by the passage of liquid vitreous through the vitreous cortex at either the prepapillary hole or the premacular cortex and into the potential space between the vitreous cortex and the ILM. The liquid vitreous then dissects and fills the potential space. The subsequent volume displacement of liquid vitreous through holes in the vitreous cortex results in vitreous collapse and a PVD. Liquid vitreous may also pass through an intact vitreous cortex which creates a slower detachment occurring as a result of a pathologic condition, such as diabetes.
Defects in the premacular vitreous cortex have been identified in autopsy eyes by Sebag and Balazs18 and confirmed by Kishi and Shimizu35 using differing techniques. The premacular or prefoveal hole appears to correspond to the posterior aspect of the posterior precortical vitreous pocket (PPVP). In this region liquefied vitreous is immediately adjacent to the vitreous cortex. It is unknown what allows the passage of liquid vitreous through the vitreous cortex to disrupt the vitreous cortex ILM adhesion, but mechanical, hormonal, and biochemical changes have been implicated.
Although vitreous detachment typically results in cleavage between the posterior vitreous cortex and the ILM of the retina, clinical and electron microscopic observations suggest that this is not always the case, particularly in younger persons and in pathologic states. Microscopic studies have confirmed the multilaminar nature of the vitreoretinal interface, including the direct insertion of vitreous cortical collagen fibers into the ILM of the retina. These insertions are particularly firm in areas where the ILM is thinnest, such as the fovea (where the ILM may measure as few as 10 nm) and the basal region (where it again thins to as few as 50 nm).6,10 In young persons, adhesions between the ILM of the retina and the vitreous cortex may be stronger than the Müller cells themselves, leading to a different plane of separation than between the ILM and posterior vitreous cortex during PVD. Vitreous cortex remnants have been identified at the fovea by scanning electron microscopy after spontaneous vitreous detachment in an autopsy series.36
In addition to the topographic and age-related differences in the vitreoretinal interface, gender-related differences in the rates of vitreous separation also exist. In most clinical series, women are twice as likely to present with symptomatic PVD37 (65%–68%) as men (32%–35%). This is paralleled by gender-related differences in disease rates associated with vitreoretinal separation. Macular holes occur between three and four times more frequently in women than in men, whereas nontraumatic giant retinal tear occurs three times more frequently in men than in women. The reasons for this disparity remain unclear, but these data may reflect gender-related differences in the strength of the vitreoretinal interface at different locations with the eye.
CELL-MEDIATED TRACTION OF THE CORTICAL VITREOUS GEL (HYPOCELLULAR GEL CONTRACTION)
Mechanical separation of the vitreous from the ILM is believed to occur in normal eyes in the absence of any cellular contribution or energy-dependent processes. Under pathologic circumstances, the cortical vitreous gel may also contract rather than separate at the vitreoretinal interface. This leads to the development of vitreoretinal traction and complex forms of retinal detachment in the presence of retinal breaks. Vitreous contraction has long been recognized as a factor in complex retinal detachments.38 However, the biologic basis for the contraction of vitreous gel was not understood until relatively recently.
Machemer39 first emphasized the critical role of cells in the genesis of vitreoretinal traction. Early histopathologic and ultrastructural studies on patients undergoing vitrectomy for PVR demonstrated transformed retinal pigment epithelial (RPE) cells, retinal glia, fibroblasts, and macrophages in membranes that were hypothesized to be contractile. It was proposed that these membranes, composed of densely packed interdigitating cells and ECM, produced tractional forces in a manner analogous to the contraction of syncytial smooth muscle elements as a result of actin and myosin filaments within the cytoplasm, particularly of myofibroblasts. However, clinical observations of the vitreous suggested that vitreoretinal traction could occur by mechanisms other than syncytial hypercellular membranes.
A different mechanism is required to explain the development of vitreous cortical shrinkage that occurs in the region of the vitreous base following vitrectomy. The ability of fibroblastic cells of different proliferative potential to contract a collagen latticework was first described by investigators evaluating the wound healing response in skin.40 Subsequent investigations in the dermatologic and ophthalmologic literature corroborated the applicability of this system for the testing of antiproliferative and anticontractile drugs.41,42 Clinical investigators pointed out the applicability of this experimental system as an explanation for contraction of the vitreous gel by dispersed intraocular cells.43,44 Various ocular cell types including dermal, scleral, or chorioretinal fibroblasts; pigment epithelial cells; and glial cells, in decreasing order of magnitude, will contract a clear gel of type I or type II collagen to a small fraction of its original volume with resultant opacification. Scanning and transmission electron micrographs of contracted gels reveal similarities with specimens of contracted vitreous taken from experimental animals and humans with PVR (Fig. 11-5). This process, termed hypocellular gel contraction, requires the presence of either serum, specific serum components, or other non–serum-derived growth factors to occur. Thrombin, transforming growth factor-β, platelet-derived growth factor, and fibronectin have all been shown to stimulate this process and can substitute for the serum requirement to variable degrees.45,46 Similarly, other pharmacologic compounds, which either intrinsically inhibit cellular contractility47,48,49,50 or interfere with cellular attachment to collagen through fibronectin-mediated binding sites51,52 can inhibit this process. Conventional and low-molecular-weight heparin also inhibit cellular attachment to collagen and, consequently, contraction. Various heparin forms also inhibit collagen polymerization.52,53 Antiproliferative compounds that innately inhibit the cellular contractility process include steroids, certain fluoropyrimidines, colchicine, and paclitaxel 53
![]() Figure 11.5. Scanning electron micrograph of contracted collagen gel. Note paucity of cellular elements despite marked compaction of collagen fibers. |
Quantitative analysis of these effects indicates that human dermal fibroblasts are nearly 20 times more potent contractors of collagen than human RPE cells. For example, 100,000 dermal fibroblasts will contract a collagen gel to <10% of its original volume within 48 hours, contrasted to a negligible change at 48 hours for 100,000 human RPE cells. However, given 120 hours, 1 million human retinal RPE cells will contract a gel to <30% of its original volume43 (Fig. 11-6). Using time-lapse video cinematography, it can be shown that RPE cells will pull in collagen fibrils in a hand-over-hand fashion at a rate of approximately 2.5 μm per minute,44 and this effect can be blocked by the addition of a peptide analogue that inhibits fibronectin-mediated binding.51 Similar effects have been demonstrated for human retinal glial cells, although the rate of contraction is less than that of either pigment epithelial cells or fibroblasts.54
In addition to demonstration of these effects in an in vitro model using purified type I and type II collagen, other investigators have demonstrated similar effects in vitro using native bovine vitreous. Although pigment epithelial cells, glial cells, and fibroblasts all produce collagen and vitreous contraction, the effects of capillary endothelial cells are modest, and no contraction is induced by either erythrocytes, blood leukocytes, or activated macrophages.55
Histologic and ultrastructural studies of contracted gels disclose consistent morphologic changes that account for these contractile events. Pigment epithelial cells cultured on plastic demonstrate a rounded or cuboidal morphology when subconfluent. It has been previously shown that when pigment epithelial cells are cultured on either vitreous, collagen, or fibrin they assume a more mesenchymal configuration, including the development of dendritic extensions and lamellipodia.56 It is believed that the development of these lamellipodia are critical for locomotion of cells and for the generation of the tractional forces. Histologic cross sections of contracted gels reveal that the cells may produce profound volumetric changes in gels without the development of typical cellular membranes. In contracted gels, cells appear to be dispersed at uniform distances with relatively infrequent cell interactions, particularly in the case of fibroblasts (Fig. 11-7). It is believed that the mechanism of contraction in these cases relates to compaction of vitreous fibrils adjacent to cells through membranous interactions between fibronectin and collagen binding sites on the cells and individual collagen fibrils and HA with resultant extrusion of water.
CLINICAL CONDITIONS OF THE VITREOUS; VITREOPATHIES
In this portion of the chapter we discuss clinical conditions that primarily involve the vitreous or in which the vitreous plays a major pathogenic role. The importance of the vitreous in a host of vitreoretinal diseases is becoming increasingly evident. These conditions will be discussed in the perspective of recent advances in vitreous anatomy, biochemistry, imaging, and pathophysiology.
DETACHMENT OF THE VITREOUS BODY
The separation of the vitreous cortex from the retinal surface is a relatively frequent and often a clinically significant occurrence. It is important because of the bothersome and at times alarming symptoms and also because of the frequent association of significant ocular pathology such as retinal tears, retinal detachment, and vitreous hemorrhage.
Incidence
Most vitreous detachments involve the vitreous posterior to the vitreous base and thus are referred to as posterior vitreous detachment (PVD). The exact incidence is unclear, although it is generally agreed that it is age related. The estimates of the occurrence of PVD in the general population vary from one series to another. The incidence in clinical studies is 53% of phakic patients older than 50 years, 65% older than 65 years, and virtually 100% in aphakic eyes.58,59 However, Foos 60 noted in a large autopsy series that the incidence of PVD was lower than in clinical studies. This is owing to difficulty in clinically identifying a PVD. For example, areas of liquefaction may simulate a PVD. In Foos’ study, PVD first occurred in the fifth decade in phakic patients, with a 17% occurrence noted by the sixth, 51% by the seventh, and 53% by the ninth decades. The incidence rose to 72% in aphakic patients. PVD is 7 to 10 times more frequent in aphakic eyes as compared with phakic eyes. This is related to mechanical changes in vitreous position in the aphakic eye and also because of biochemical changes. Surgical aphakia results in anterior displacement of the vitreous during ocular movements. Aphakia with extracapsular cataract extraction (ECCE) has a lower incidence of PVD than with intracapsular cataract extraction (ICCE), if the posterior capsule is intact.61 This is probably because the intact posterior capsule prevents anterior vitreous displacement.
Aphakia also alters the biochemical profile of the vitreous. HA concentration decreases following ICCE, resulting in vitreous liquefaction.62 ECCE results in a minimal decrease in HA concentration compared with ICCE.63 However, even with modern surgery techniques, PVD is common. In one series of eyes undergoing small-incision phacoemulsification with posterior chamber intraocular lens implantation, PVD developed in 21% at one week, 31% at 1 month, and 59% at one year.64
The changes in the incidence of PVD with aphakia after ICCE and ECCE parallel the incidence of retinal detachment following ICCE (3%–7%) and ECCE (0.5%–2%).65 This is because the formation of retinal breaks and subsequent retinal detachment is dependent on the formation of liquid vitreous and an abnormal vitreoretinal adhesion, which create an anomalous PVD.66 Liquid vitreous and subsequent PVD increase vitreoretinal traction in the region of the vitreous base, resulting in retinal tears.67 Liquid vitreous then passes through these tears to dissect the retina off the RPE cells. PVD is more common in females.37,67 It occurs an average of 10 years earlier in myopic patients when compared with emmetropic or hyperopic patients.58,59
The incidence of partial PVD appears to be very low, which supports the concept that the process, once begun, usually proceeds to completion in a relatively short period in the absence of abnormal vitreoretinal adhesion. However, an incomplete PVD can remain unchanged for long periods. When a partial PVD is present, it is usually found in the upper portion of the vitreous body or at the macular or optic nerve. Linder58 evaluated 115 patients clinically with symptoms of the sudden appearance of floaters with or without light flashes and found that 95% demonstrated a complete PVD with collapse, whereas 4% had a superior vitreous detachment and the remaining 1% showed a funnel-like vitreous detachment with attachment remaining at the disc. The two types of partial vitreous detachment were noted to convert to total posterior detachment in most cases. Jaffe59 noted a similar incidence, with 82 of the 84 patients who presented with symptoms of suddenly appearing entopsias demonstrating a PVD with collapse.
Signs and Symptoms
The subjective symptoms of a PVD are varied, and many patients may have a vitreous detachment without being aware of its occurrence. In Linder’s58 series of acute PVDs, approximately 25% of the cases had an unrecognized PVD in the fellow eye. The most common symptom is the sudden onset of floaters with or without light flashes. Patients who present with floaters and flashes appear to be at higher risk for a posterior vitreous separation than those patients who present solely with light flashes or floaters.67 Two theories concerning the cause of light flashes have been proposed. One theory suggests that vitreous traction on the retina at the site corresponding to a retinal break causes symptoms, whereas the second theory suggests that during ocular movement a detached vitreous affects the retina causing the light flashes.68,69
Floaters may take many forms and are most easily noted by the patient when viewed against a light background or in bright sunlight. Floaters may be present in a liquefied vitreous without a PVD, but the sudden onset of floaters is suggestive of an acute change in the status of the posterior vitreous. This common symptom of an acute PVD may represent condensed vitreous fibrils, vitreous blood, or, in the case of a single large floater, glial tissue on the posterior surface of the vitreous cortex. In most cases, visual acuity is quantitatively unaffected by the PVD. However, if accompanied by significant hemorrhage, a posterior vitreous separation may result in a marked decrease in visual acuity or blurring, dependent on the degree of hemorrhage.
When the vitreous detaches from the area of the optic nerve head, a tag of tissue may be avulsed, and at times a complete ring of peripapillary tissue may come forward with the vitreous. The presence of this ring is pathognomonic for a PVD (Fig. 11-8). Electron microscopic studies on autopsy eyes with PVDs demonstrate frequent glial epipapillary membranes on the vitreous side of the ILM of the optic nerve and surrounding retina.70,71 There are interruptions in the epipapillary membranes, as well as separations of the membranes from the underlying ILM of the disc, both of which contain incarcerated collagen fibers identical with vitreous fibrils. This intimate association of the vitreous in the disc area accounts for the frequent avulsion of tissue in a PVD. Glial tissue occurs on the posterior hyaloid in 57% of PVDs.
Pigmented vitreous cells may also be seen on slitlamp biomicroscopy in the presence of a PVD. The presence of these pigmented cells in the anterior vitreous cavity is highly associated with a finding of a peripheral retinal tear.72,73,74 Careful examination of the retinal periphery with scleral depression and/or contact lens is necessary because of the significant association between the cells and peripheral retinal tears. Vitreous hemorrhage may also be present, obscuring the view of the retina. These patients are also at very high risk of having a retinal break as the cause of their dispersed hemorrhage.
Complications
The cortical vitreous is more firmly attached to the ILM in the area of the vitreous base, optic nerve, macula, and retinal blood vessels. Separation of the vitreous may transmit traction to these attachments, leading to vitreous hemorrhage or retinal tears. Persistent vitreous traction on retinal tears is an important factor in the development of a retinal detachment. Following acute PVD, retinal tears are detectable clinically in 8% to 48% of eyes.37,58,59,74,75
In a meta-analysis of 1,568 patients with acute symptomatic PVD, there was a retinal tear present in 22% on initial examination.74 Pigmented cells in the anterior vitreous, vitreous hemorrhage, or retinal hemorrhage are associated with initial retinal breaks. The occurrence of delayed retinal breaks, defined as at least 1 month after initial examination, is approximately 2%. Delayed retinal breaks after PVD are associated with new symptoms, vitreous hemorrhage, or peripheral retinal hemorrhage. Patients with these findings, lattice degeneration, or high myopia have a higher risk for retinal breaks and retinal detachment and may warrant closer follow-up than patients without these risk factors.74,76
Vitreous hemorrhage from PVD may originate from vessels on the optic disc or retina. These hemorrhages are present in 13% to 19% of eyes with PVD.58,59,75 Hemorrhage may preclude an adequate retinal examination, which is essential to determine the presence or absence of a retinal tear. A careful fundus examination with scleral depression and contact lens biomicroscopy is required, because roughly two thirds of the eyes with acute PVD and vitreous hemorrhage also have retinal breaks.59,75,77
In an autopsy series, Spencer and Foos78 also noted a high incidence of firm, paravascular vitreoretinal attachments. In 25% of the eyes, with an attached posterior vitreous, this led to paravascular retinal rarefaction with cystic spaces adjacent to blood vessels in the inner retinal layers. In addition, there was a connection between the ILM and the retinal blood vessels, as well as an irregularity of the ILM suggesting vitreous traction. Paravascular retinal tears, on the other hand, occurred in 11% of the eyes, and of these all had PVDs. Both types of retinal pathology were usually seen after the age of 50 years. It is, therefore, not surprising that retinal and vitreous hemorrhage following a PVD is so frequently associated with a retinal tear.
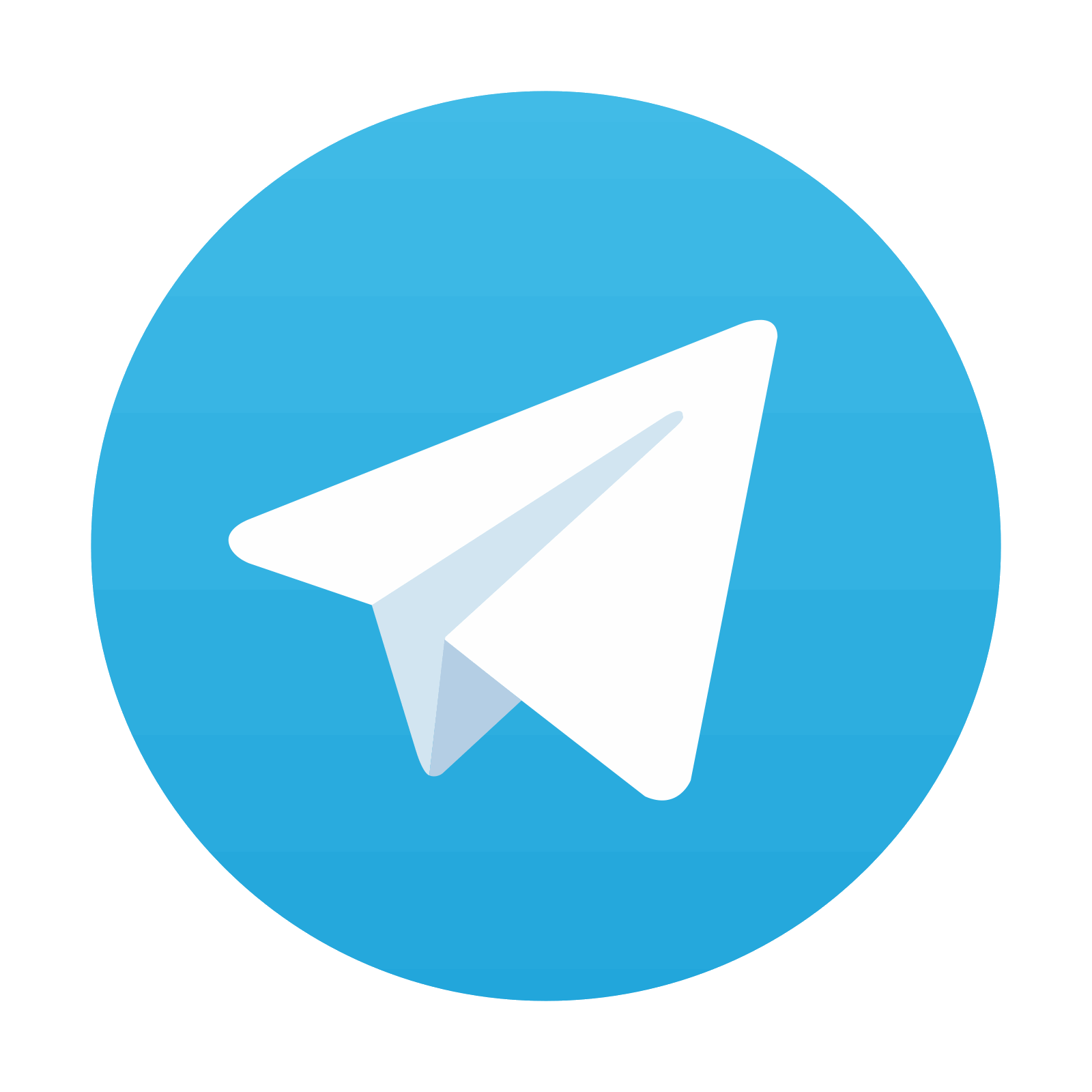
Stay updated, free articles. Join our Telegram channel
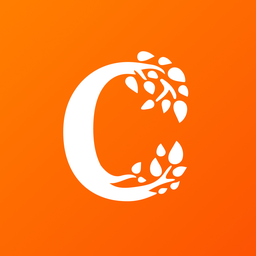
Full access? Get Clinical Tree
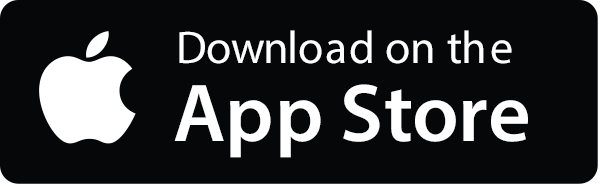
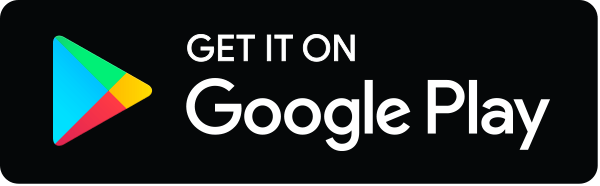
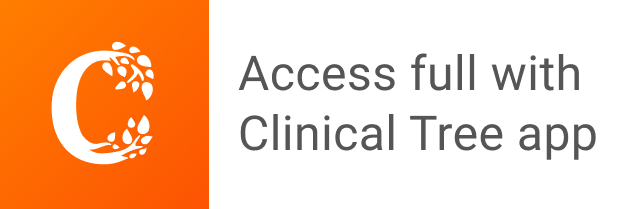