Fig. 13.1
Subcellular planar polarity. (Modified from Deans [2])
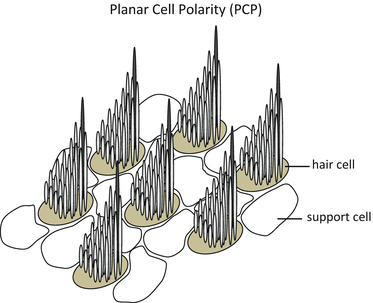
Fig. 13.2
Planar cell polarity (PCP). (Modified from Deans [2])
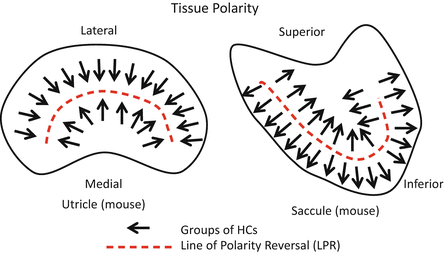
Fig. 13.3
Tissue polarity. (Modified from Deans [2])
13.4.1 Subcellular Planar Polarity
At E12.5, the first HCs can be distinguished, and a single cilium emerges from the center of the cell surface. The cilium is surrounded by elongated microvilli [42, 43], and it lengthens to form the kinocilium to subsequently establish subcellular planar polarity as the cilium migrates to one side of the apical cell surface.
Polarization of the stereociliary bundle is cell intrinsic and occurs independently from mechanisms directing planar polarity at the level of PCP or tissue polarity. As described below, auditory and vestibular HCs in vangl2 knockout [44] and frizzled 3/6 double knockout mice [45] are incorrectly oriented relative to the neighboring cells, although their mutant HCs still form polarized stereociliary. Fewer mutations only affect subcellular planar polarity, while a conditional knockout mouse disrupting the function of IFT88/Polaris is an essential factor for intraflagellar transport and cilium formation [46]; protocadherin 15 mutants [47] have been reported to disrupt subcellular planar polarity construction.
Some of the molecular signals that direct formation of the polarized bundles are beginning to be uncovered. The function of P21-activated kinase PAK asymmetrically localized in the vicinity of the kinocilium [48] is further regulated by the small GTPase Rac1 which is itself a well-characterized regulator of actin dynamics [49]. GTPase Rac1 is, in fact, involved in certain functions of P21-activateed kinase PAK. The Rac1-PAK signaling pathway is also dependent upon the function of Kif3a. The Rac1-PAK signaling pathway is also dependent upon the function of Kif3a [50], a component of the Kinesin II motor complex that is necessary for plus-end microtubule trafficking and anterograde intraflagellar transport [51]. However, Kinesin II, whose identity is still unknown, is believed not to be the motor factor that translocates the kinocilium.
13.4.2 Planar Cell Polarity and the Coordinated Orientation of Adjacent HCs
Many essential molecules regulating this level of planar polarity have been identified through genetic screening in Drosophila, including the core PCP proteins Frizzled, Dishevelled, Van Gogh, Prickle, Diego, and Flamingo. The subcellular distributions of core PCP proteins are likely conserved between the mouse and Drosophila at the line of polarity reversal (LPR). Core PCP proteins are asymmetrically localized in the epidermal cells of developing Drosophila wings with Frizzled and Dishevelled enriched at the distal cell boundary near the site of hair formation and Van Gogh and Prickle enriched at the proximal side. Flamingo is present at both cell boundaries. In the developing mouse utricle the core PCP proteins Frizzled6 (Fz6) and Prickle-like 2 (Pk2) are located on opposite sides of HCs and supporting cells: a pattern similar to the relative distribution of Frizzled and Prickle in Drosophila, suggesting that other PCP proteins are similarly distributed. The remaining core PCP proteins in mice are asymmetrically localized at the cell boundaries; however, proximal versus distal distributions have not been established. Pk2 is enriched at the same side of vestibular HCs, regardless of the stereociliary bundle orientation or cellular position relative to the LPR [52–56].
13.5 Innervation of Vestibular Hair Cell
Each vestibular afferent neuron has its cell body in the cochleovestibular (CV) ganglion and sends a peripheral axon toward the vestibular sense organs and a central axon to the vestibular nuclei in the brainstem. The CV ganglion consists of two cell clusters extending in a rostral–caudal direction in the internal auditory canal. It consists of a superior and inferior cell group related to the superior and inferior divisions of the vestibular nerve trunk, respectively. The superior division supplies the cristae of the superior and lateral canals, the macula of the utricle, and the anterosuperior part of the macula of the saccule. The inferior division supplies the crista of the posterior canal and the main macular portion of the saccule. The inferior division supplies the crista of the posterior canal and the main portion of the macula of the saccule. Medial to the vestibular ganglion, the nerve fibers of both divisions merge to form a single trunk, which enters the brain stem [57].
As one of the cranial peripheral nerves, the CV nerve is composed of both neurons and glia [58]. These two cell types arise developmentally from distinct sources, viz., the glial cells derived from neural crest cell (NCC) progenitors [59–61], while the neurons originate almost exclusively from the otic placode [59, 62, 63].
Apart from projecting central axons to the hindbrain, CV neurons also project peripheral neuritis to sensory targets developing within the otic epithelium [64]. The glial cells of the CV nerve are derived from NCCs that emigrate from the hindbrain at the level of rhombomere 4 (R4).
CV neurons extend peripheral neuritis to the developing vestibular sensory epithelium as early as E11.5 and extend central axons to the hindbrain as early as E12.5 [64, 65] and release some important factors related this neural development. In mice, disturbance of ERBB2 (a receptor that mediates neuron–glia interactions via the ligand Neuregulin1 [66]) compromises development of the CV nerve. Loss of ErbB2 results in altered migration of CV neuronal cell bodies, abnormal targeting of CV peripheral neurites, and reduced number of CV neurons [67]. Molecularly blocking Semaphorin/Neuropilin signaling in chicks disrupts NCC migratory pathways and impairs the inward movement of epibranchial placodal neurons [68]. In certain studies, ablation of NCC migration by physical or molecular methods in chicks results in reduced numbers of neuroblasts migrating from the epibranchial ganglia and abnormal projection of central axons [69–71].
The maculae contain two types of HCs that are distinguished from each other by the synaptic structure formed with the afferent neurons. Type I HCs receive large calyx nerve endings that surround the cell body, while type II HCs make bouton-like contacts with nerve endings. The two HC classes are innervated by three types of afferent neurons. The calyx-only class of neurons contacts clusters of type I HCs in the striola region. Dimorphic neurons contact HCs throughout the sensory epithelia, forming calyxes with type I HCs and boutons with type II HCs. Bouton-only afferents only contact type II HCs located outside the striola. Each class of afferent neurons may contact multiple HCs, and the dimorphic and bouton-only neurons contact groups of HCs located on either side of the LPR. Despite the range of bundle orientations present in the utricle, afferent neurons only contact HCs with similar stereociliary bundle orientations. Although developmental mechanisms coordinating neuronal innervation and stereociliary bundle orientation are not well known, there is a single cell type that morphologically resembles the mature type II HCs prior to the obvious distinction of the two HC types. From the later appearance of morphologically distinct type I HCs during regeneration in the avian utricle, it has been suggested that type I HCs differentiate from the type II HCs in a serial progression of development. However, earlier studies of spatiotemporal patterns of HC birth have suggested that HCs in regions where type I HCs predominate in the mature sensory epithelium are born first, while hair cells in those regions where type II HCs predominate are born later, indicating that types I and II cells may be birth-specific.
References
1.
Wu DK, Kelley MW. Molecular mechanisms of inner ear development. Cold Spring Harb Perspect Biol. 2012;4(8):a008409. doi:10.1101/cshperspect.a008409.PubMedCentralPubMed
2.
Deansa MR. A balance of form and function: planar polarity and development of the vestibular maculae. Semin Cell Dev Biol. 2013;24(5):490–8. doi:10.1016/j.semcdb.2013.03.001.
3.
Riccomagno MM, Takada S, Epstein DJ. Wnt-dependent regulation of inner ear morphogenesis is balanced by the opposing and supporting roles of Shh. Genes Dev. 2005;19(13):1612–23.PubMedCentralPubMed
4.
Acampora D, Merlo GR, Paleari L, Zerega B, Postiglione MP, Mantero S, Bober E, Barbieri O, Simeone A, Levi G. Craniofacial, vestibular and bone defects in mice lacking the Distal-less-related gene Dlx5. Development. 1999;126(17):3795–809.PubMed
5.
Depew MJ, Liu JK, Long JE, Presley R, Meneses JJ, Pedersen RA, Rubenstein JL. Dlx5 regulates regional development of the branchial arches and sensory capsules. Development. 1999;126:3831–46.PubMed
6.
Merlo GR, Paleari L, Mantero S, Zerega B, Adamska M, Rinkwitz S, Bober E, Levi G. The Dlx5 homeobox gene is essential for vestibular morphogenesis in the mouse embryo through a BMP4-mediated pathway. Dev Biol. 2002;248(1):157–69.PubMed
7.
Hadrys T, Braun T, Rinkwitz-Brandt S, Arnold HH, Bober E. Nkx5-1 controls semicircular canal formation in the mouse inner ear. Development. 1998;125(1):33–9.PubMed
8.
Wang W, Van De Water T, Lufkin T. Inner ear and maternal reproductive defects in mice lacking the Hmx3 homeobox gene. Development. 1998;125(4):621–34.PubMed
9.
Urness LD, Paxton CN, Wang X, Schoenwolf GC, Mansour SL. FGF signaling regulates otic placode induction and refinement by controlling both ectodermal target genes and hindbrain Wnt8a. Dev Biol. 2010;340(2):595–604. doi:10.1016/j.ydbio.2010.02.016.PubMedCentralPubMed
10.
Brown AS, Epstein DJ. Otic ablation of smoothened reveals direct and indirect requirement for Hedgehog signaling in inner ear development. Development. 2011;138(18):3967–76. doi:10.1242/dev.066126.PubMedCentralPubMed
11.
Liang JK, Bok J, Wu DK. Distinct contributions from the hindbrain and mesenchyme to inner ear morphogenesis. Dev Biol. 2010;337(2):324–34. doi:10.1016/j.ydbio.2009.11.001.PubMed
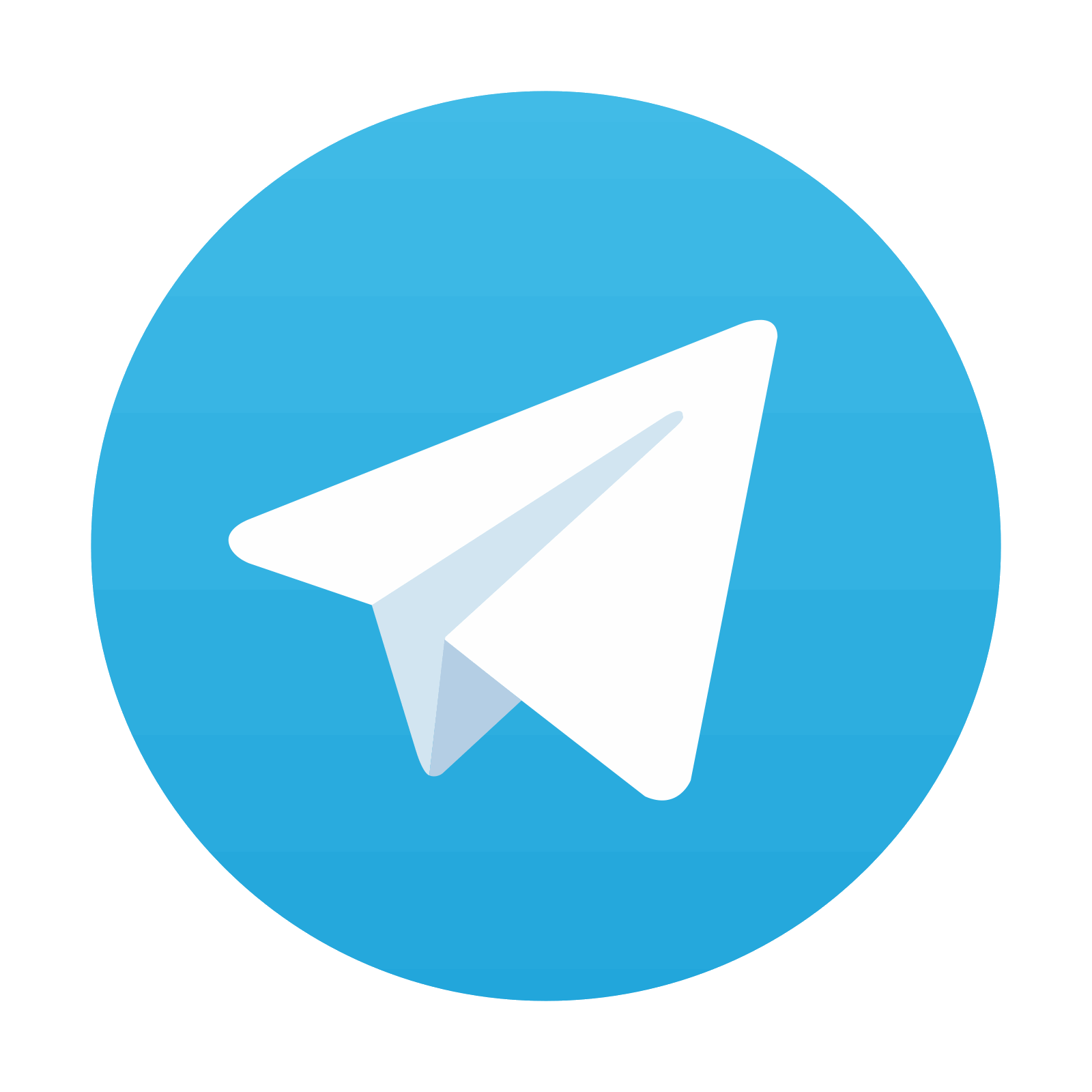
Stay updated, free articles. Join our Telegram channel
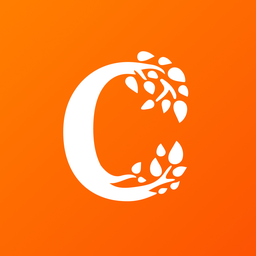
Full access? Get Clinical Tree
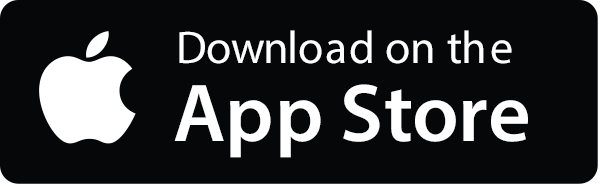
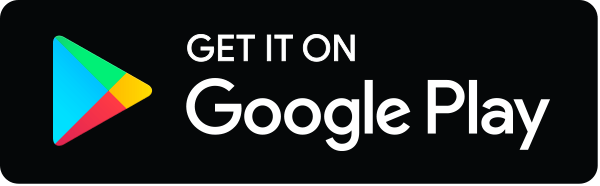