Fig. 1.1
Autofluorescence imaging of the retinal pigment epithelium (RPE), demonstrating the topographic distribution of fluorescence in the posterior segment of the human eye. Many fluorophores are contained within RPE, so that areas of normal RPE may exhibit autofluorescence compared to dark areas of patchy RPE atrophy
Fluorescein angiography (FA) is a technique used to image blood flow of the retina and choroid by using sequential fluorescence imaging following the intravenous injection of sodium fluorescein (Fig. 1.2). Histopathology studies have revealed that there is an accumulation of autofluorescent material in the retinal pigment epithelium as well as autofluorescent deposits of extracellular material in macular and retinal disease [146]. Use of fluorescein angiography allows for the visualization of atrophic patches that appear well-demarcated, hyperfluorescent areas due to loss of retinal pigment epithelium [148]. These cells, if intact, would otherwise weaken transmission.
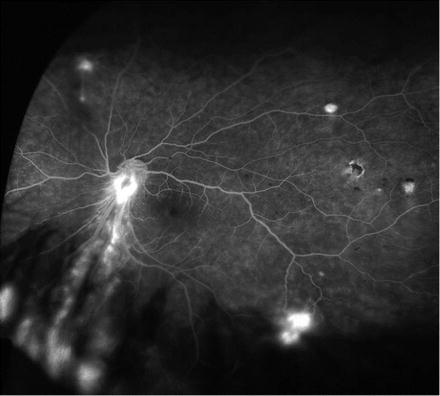
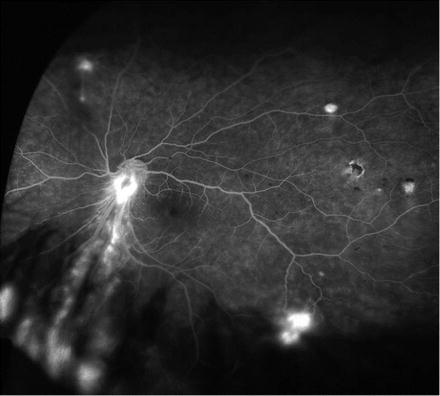
Fig. 1.2
Wide field retinal angiography to image blood flow of the retina and choroid obtained after intravenous injection of sodium fluorescein. In addition to monitoring for non-perfusion, angiography can be used to detect angioma (shown here) or vascular leakage, which can be a sign of graft rejection
Indocyanine green (ICG) is a cyanine dye that allows for enhanced imaging patterns of circulation when compared to fluorescein dye given a spectral absorption between 805 and 835 nm [149]. As with fluorescein dye, indocyanine allows for the visualization of atrophic areas of degeneration. Indocyanine green has primarily been used in the diagnosis and interpretation of occult choroidal neovascularization in age-related macular degeneration and for identification of angiomatous lesions of the retina and polyps in the choroid [150]. The unique properties of indocynanine green allows for visualization of macular dystrophies through overlying pathologic conditions such as hemorrhage, serous fluid, lipid, and pigment [151]. Indocyanine green has been utilized as an adjunct tool along with fluorescein angiography for the diagnosis of age-related macular degeneration [151].
Angiography allows the ophthalmologist to discern the perfusion status of the retina and choroid, as diseases that impair perfusion can be diagnosed on the basis of abnormalities in the dye filling pattern on sequential angiogram photos. In addition to monitoring for non-perfusion, FA and ICG angiography can be used to monitor for vascular leakage, which can be diagnosed on the basis of accumulation of extracellular dye due to increased vascular leakage. There are several reasons why this is particularly useful to the field of stem cell transplantation. First and foremost, in the absence of native RPE there is secondary non-perfusion of the choriocapillaris, and this non-perfusion is evident on both fluorescein and ICG angiography. Animal studies suggest that the choriocapillaris can reperfuse after replacement of the RPE, and thus angiography can be used to monitor the outer retinal blood supply for the success of transplanted cells. Both techiques allow for the monitoring of vascular integrity (Fig. 1.2) [150, 152]. In addition ICG and FA allow the ophthalmologist to detect leakage of dye, which can be a sign of graft rejection.
Optical coherence tomography (OCT) is an imaging method currently in widespread clinical use that provides in vivo images from the human retina. OCT relies on differences in the index of refraction of ocular tissue to generate a cross-sectional image of the retina and the vitreoretinal interface. OCT can be used to measure foveal and extrafoveal retinal thickness and can be used to determine the thickness of the outer nuclear layer and integrity of the outer segment–inner segment junction. OCT can be used to detect RPE atrophy and outer retinal atrophy and to determine the thickness of the nerve fiber layer. In geographic atrophy, OCT can reveal atrophy of the choriocapillaris, particularly with enhanced depth choroid imaging. In the study done by Neurotech on ciliary neurotrophic factor (CNTF), OCT was used to demonstrate increasing thickness of the outer retina in patients with nonexudative AMD who received the CNTF-releasing implant [66]. In principle, OCT can be used to monitor the ability of transplanted stems cells to repopulate Bruch’s membrane, the return of retinal thickness back to normal, and reestablishment of choroid thickness in patients with atrophy. OCT can also be used to determine adverse events after transplantation, including the development of retinal edema, after treatment of patients with exudative and nonexudative age-related macular degeneration (Fig. 1.3) [141, 153, 154].
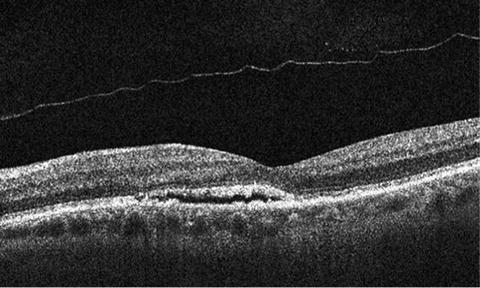
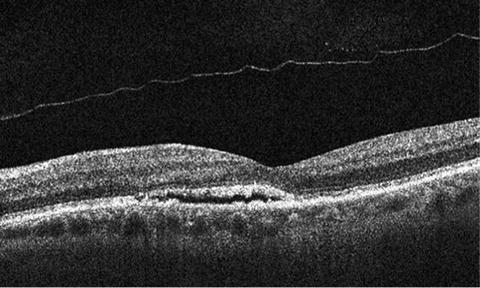
Fig. 1.3
Optical coherence tomography (OCT) reveals a small pocket of subretinal fluid in an asymptomatic patient with age-related macular degeneration. OCT can be used to measure the thickness of the outer nuclear layer and integrity of the outer segment–inner segment junction after successful cell transplantation
In addition to these structural studies, there are several excellent functional tools for determining retinal function in eyes of retinal degenerations treated with stem cells. Microperimetry can assess macular sensitivity and retinal fixation by providing a retinal visual function map on a selected, localized fundus location with preset or customized scan patterns (Fig. 1.4a, b) [155]. In this technique, the retina is stimulated by illumination with small spot sizes under direct visualization; this allows the examiner to discern the retinal sensitivity as a function of illumination level and spot size in areas of the retina affected by retinal degenerations, and in treated and control regions. In principle, a beneficial effect of transplantation would be manifested by increasing retinal sensitivity on microperimetry.
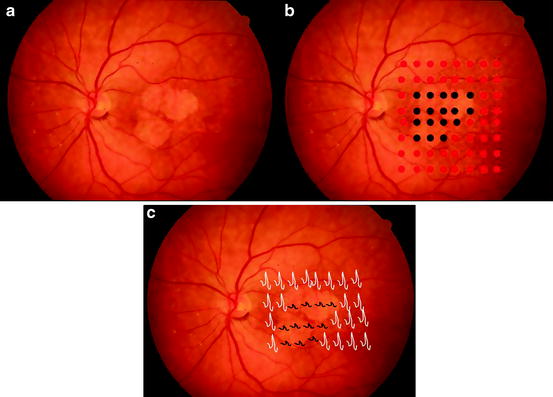
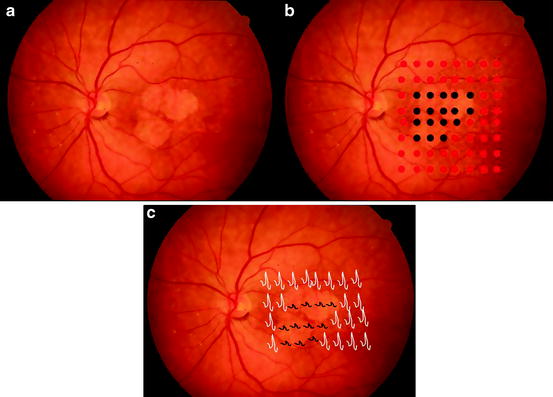
Fig. 1.4
(a) Microperimetry can be used to assess macular sensitivity and retinal fixation in normal and atrophic areas of retina by providing a retinal visual function map on a selected, localized fundus location with preset or customized scan patterns. (b) In this technique, the retina is illuminated with small spot sizes under direct visualization; this allows the examiner to demonstrate decreased retinal sensitivity in regions of geographic atrophy (Right panel, black circles) and normal sensitivity in adjacent regions (Right panel, red circles). (c) Multifocal electroretinography (mERG), which allows for topographical measure of electrical activity in distinct areas of the retina, can be used to monitor disease progression and efficacy of therapy. In retinal degenerations, there is typically a decrease in amplitude, or absent ERG signal, in areas of retinal dysfunction (black tracings)
Similarly, multifocal ERG can also be used to determine a decrease in retinal function due to disease, and an improvement in dysfunction after retinal transplants. Multifocal ERG allows for a topographical measure of electrical activity in distinct areas of the retina (Fig. 1.4c) [156]. Multifocal ERG can stimulate multiple retinal areas at the same time and detect each response independently [157]. In retinal degenerations, there is typically a decrease in amplitude, or absent ERG signal, in areas of retinal dysfunction; this change is often present only in an area of dysfunction. There is an improvement in the global ERG in animals with retinal degenerations receiving transplants of stem cells (Fig. 1.5) [158]. In principle, improvement in retinal function after stem cell transplantation should be topographic and result in a focal improvement in ERG in the area of the transplant. These advantages in imaging and focal detection of function should not be overlooked, since cell transplants in other areas of the body do not have similar advantages. These advantages present a unique opportunity to detect the beneficial effects of stem cells in the treatment of retinal diseases. Their use also makes diseases such as age-related macular degeneration an attractive option to begin clinical trials with stem cells. The ranges of clinical and diagnostic tools also help provide the necessary efficacy and safety data to move trials forward [159].
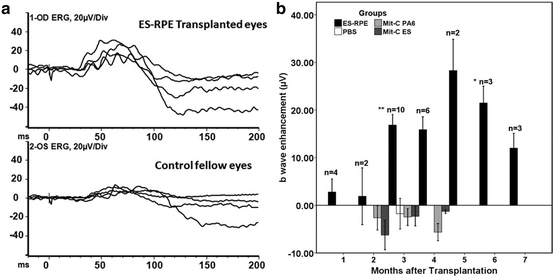
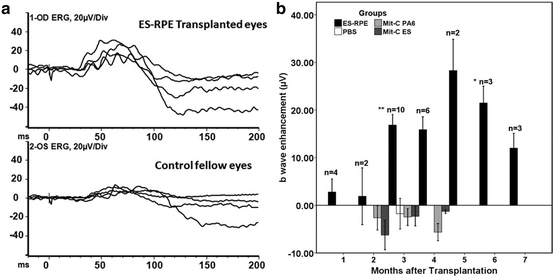
Fig. 1.5
ERGs of Rpe65 rd12 /Rpe65 rd12 mice after subretinal transplantation with ES cell-derived retinal pigment epithelial (RPE)-like cells confirm functional rescue. (a) ERG from mice after 3 months transplantation. Eyes transplanted with ES cell-derived RPE-like cells (upper) showed higher b-wave amplitudes compared with control fellow eyes (lower). Traces represent readings from different mice. (b) b-wave enhancement in mice 1–7 months post-transplantation, as indicated by black solid bars. b-wave enhancement is defined as the difference in maximum ERG responses of transplanted and control fellow eyes (μV). Unpaired t tests were performed for paired differences in b-wave peaks between transplanted and control eyes. At 3 and 6 months post-transplantation, ERGs from transplanted eyes show a statistically significant rescue effect (**P = 0.001 and *P = 0.038, respectively). Although the difference was not statistically significant at 4, 5, and 7 months after transplantation, the b-wave amplitudes in the transplanted eyes were consistently higher than the control fellow eyes. The number of mice analyzed per time point is indicated. ERGs were performed on both eyes (injected and control) simultaneously. There is no statistically significant difference between injected and control eyes in the other three control groups. White bar, b-wave enhancement in PBS injected mice; light-shaded bar, b-wave enhancement in mitomycin-C treated PA6 cell transplanted mice; and dark-shaded bar, b-wave enhancement in mitomycin-C treated undifferentiated ES cell transplanted mice. ES-RPE ES cell-derived RPE-like cells, Mit-C mitomycin-C, ES embryonic stem, PBS phosphate-buffered saline. Reproduced from Wang et al. [158]
Status of Efforts to Differentiate Stem Cells into Photoreceptors and RPE
As a general principle, patients who could directly benefit from cell-based therapies with retinal degenerative disease such as RP and AMD will require replacement of lost photoreceptor cells, RPE, and possibly choriocapillaris [160]. Korte et al. have shown the choriocapillaris can regenerate if areas of absent RPE can be repopulated with new RPE. Thus the clinical need here is to promote the differentiation of stem cells into photoreceptors (rods and cones) and RPE.
In dry AMD, loss of vision arises from loss of RPE and photoreceptors with secondary atrophy of choriocapillaris. A potential treatment for AMD and inherited disease that affect the RPE and photoreceptors would be cell replacement therapy, but one significant hindrance to the clinical use of cell transplantation for treatment of retinal degenerations is the availability of a source of replacement cells. Although RPE derived from prenatal and postnatal tissue has been isolated and induced to grow in vitro, such sources are limited and vary in terms of quality and expansion capacity [141, 161–163]. Moreover, it has been demonstrated that postmitotic photoreceptor precursor cells can be derived from tissue of the early postnatal mouse retina (P1–P5) [164, 165]. However, equivalent retinal cells in humans would have to be derived from second-trimester fetuses. While these studies provide solid evidence that transplantation strategies show great potential, an approach such as this would have ethical implications as well as the problem of a limited reservoir of donor cells [165].
Stem cells are an excellent source of cells for replacement therapy given the limited reservoir of donor cells for RPE replacement strategies, lack of regeneration of photoreceptors, and variation in success of autografts. Stem cells have been isolated from a variety of sources including embryo and adult eye [164, 166]. Human embryonic stem cells (hESC) are being investigated as a potential source of photoreceptors and RPE and are promising candidates for therapeutic use.
As mentioned, the strategy in cell replacement therapy using stem cells is to differentiate these cells into photoreceptors or RPE. The extracellular environment plays a critical role in the differentiation of stem cells into the target cell type and extracellular matrix can differentiate cells into the adjacent cell layer. For example, hESCs cultured on a monolayer of cells derived from mouse calverium can be induced to a neural fate and express neural progenitor markers such as paired boxed protein (PAX)-6, neurofilament, and glial fibrillary acidic protein (GFAP) (Fig. 1.6) [167]. Similarly, hESCs cultured on a monolayer of RPE (ARPE19) cells can be induced to express neural retinal markers such as vimentin, neurofilament, and cone–rod homeobox (CRX), which is essential in early photoreceptor development (Fig. 1.7) [167]. Varying extracellular matrix environs such as laminin, matrigel, and/or vitronectin and fibronectin can also induce embryonic stem cells toward a neural progenitor or RPE fate. Embryonic stem cells cultured on laminin, vitronectin, and fibronectin can be induced to express neural progenitor markers such as neurofilament and neural retina-specific leucine zipper (NRL), an intrinsic regulator of photoreceptor development (Fig. 1.8). These cells can also be induced to express RPE markers such as tight junction protein ZO-1 and bestrophin when cultured on matrigel (Fig. 1.9). Stem cells grown on human Bruch’s membrane have also been induced to differentiate to RPE (Fig. 1.10) [167].
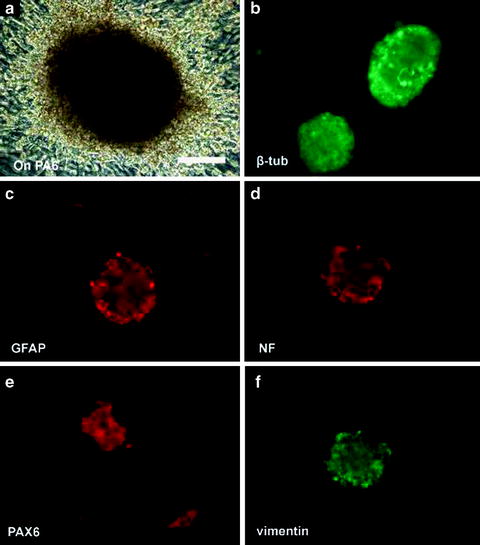
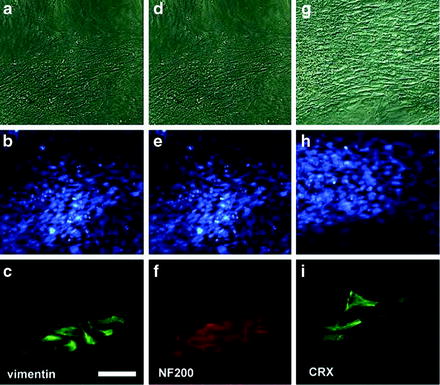
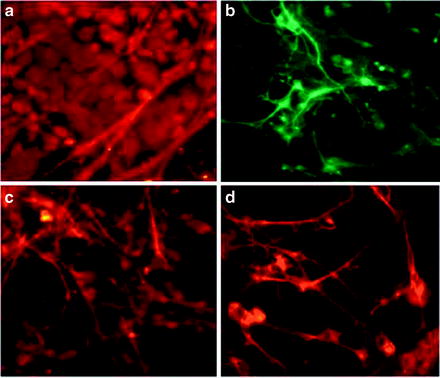
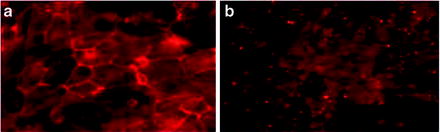
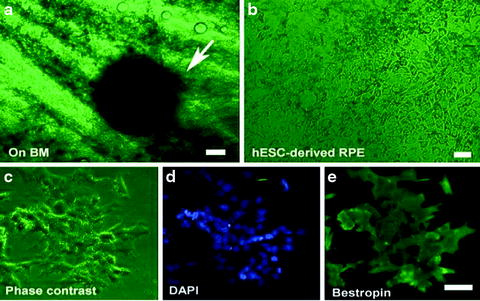
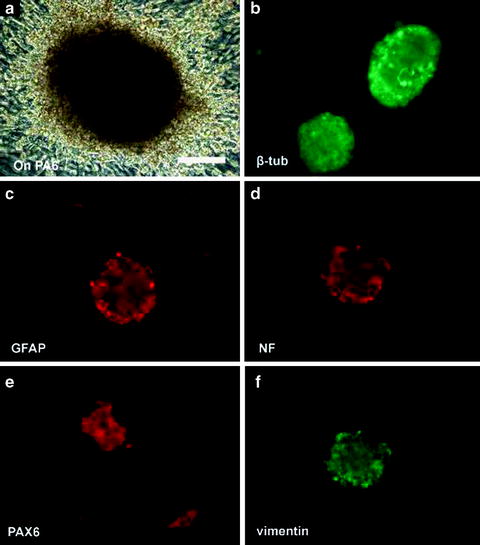
Fig. 1.6
Expression of neural progenitor markers after culturing human embryonic stem cells on mouse PA6. (a) Human embryonic stem cells became multilayered and formed pigmented spheres after culturing on mouse PA6 cells for 13 days. Immunofluorescence staining of the spheres demonstrated the presence of several neural progenitor markers including β-tubulin III (>88%) (b), GFAP (c), neural filament NF200 (>90%) (d), PAX6 (>88%) (e) and vimentin (f). Bar = 100 μm
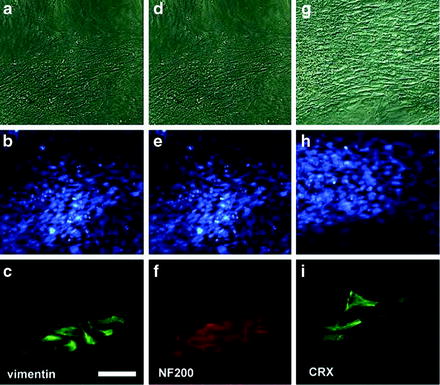
Fig. 1.7
Generation of retinal precursors from neural progenitors after culturing human embryonic stem cells on ARPE19 cells for 10 days. Top row, phase-contrast micrographs; middle row, nuclei in both ARPE19 and progenitors stained with DAPI. Progenitors expressed neural progenitor marker vimentin (C) and neural filament 200 (F) and photoreceptor-specific protein CRX (I), which is essential during early photoreceptor development. Bar = 50 μm
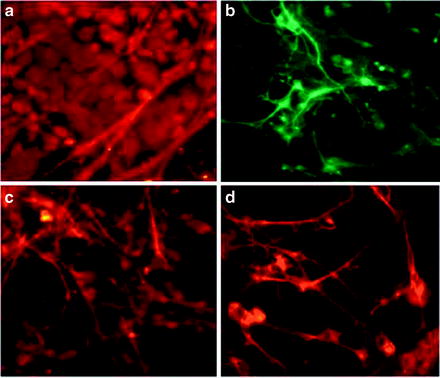
Fig. 1.8
Expression of neural progenitor markers after culturing mouse embryonic stem cells on poly-d-lysine, laminin, vitronectin, and fibronectin. Cells expressed photoreceptor marker NRL (a) and neural progenitor markers β-tubulin (b) neurofilament 200 (c) and vimentin (d)
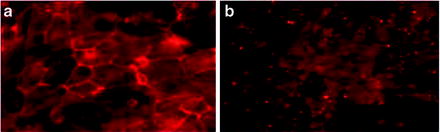
Fig. 1.9
Expression of retinal pigment epithelium (RPE) markers after culturing mouse embryonic stem cells on matrigel. Cells expressed RPE makers Z0-1 (a) and Bestrophin (b)
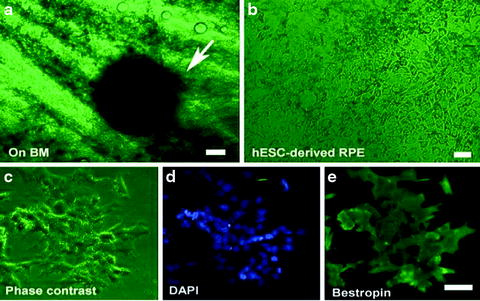
Fig. 1.10
Induction of RPE markers in human embryonic stem cells cultured on human Bruch’s membrane. (a) Cluster of pigmented human embryonic stem cells 4 days after growing on human Bruch’s membrane explants (arrow). (b) Phase-contrast micrograph of flattened pigmented epithelium-like cells on human Bruch’s membrane. (c)–(e) hESC-derived RPE under phase contrast, DAPI and Bestrophin staining, respectively. Bar = 50 μm in (a), (c)–(e); bar = 20 μm in (b)
Current Status of Human Stem Cells in Clinical Trials
Current clinical trials are underway to evaluate the potential of stem cell therapy in humans. Advanced Cell Technology, Inc. initiated a phase 1 clinical trial in humans in 2011 for the treatment of retinal degenerative disorders [141]. This is the first FDA approved trial for the treatment of macular degeneration using RPE derived from human embryonic stem cells. RPE cells were derived from a single donor human embryonic stem cell line. The preliminary report details the phase 1 trial being conducted to test the safety and tolerability of hESC-RPE in patients with advanced-stage Stargardt’s macular dystrophy and dry age-related macular degeneration. Briefly, a human embryonic stem cell (MA09) line was first used to generate hESC-derived RPE, which were then characterized and tested for pathogen contamination. After pars plana vitrectomy, submacular injections of 50,000 cells were used to treat the patient. Patients were immune suppressed with low-dose tacrolimus and mycophenolate mofetil 1 week before surgery and continued for 6 weeks postsurgery. After 6 weeks patients discontinued tacromilus and continued a mycophenolate regimen for 6 more weeks. Both patients tolerated the injection without signs of postoperative inflammation, rejection, or tumorigenicity at time of the report (4 months follow-up). In the patient with Stargardt’s macular dystrophy, transplanted cells attached to Bruch’s membrane and persisted throughout the observation period; there was possible visual improvement in the injected eye as shown by visual acuity and Goldmann visual field test. In the AMD patient no clinically detectable sign of successful transplantation was observed, although the patient did not comply with the immunosuppressive drug regimen [168]. Interestingly, there was mild visual improvement in both eyes of the patient with AMD as shown by visual acuity and Goldmann visual field test. It is unclear whether these visual improvements are due directly to the cell transplant or a secondary cause such as the immunosuppressive drugs or a placebo effect [141]. Nevertheless, these important studies demonstrate that stem cells can be transplanted into the subretinal space in humans without abnormal proliferation, teratoma formation, graft rejection, or other untoward pathological reaction or safety signal.
These short-term results in only two patients, are preliminary but provide valuable proof-of-concept evidence for future treatment of macular degeneration and Stargardt’s disease in humans. Long-term follow-up in a larger cohort of patients is needed to draw more meaningful conclusions from this trial [168]. Further trials are also needed to determine the optimal number of transplanted cells, immunosuppression regimens, and disease stage for transplantation [168].
Induced Pluripotent Stem Cells as a Therapeutic Option
The recent development of induced pluripotent stem (iPS) cells holds great promise as a potential reservoir of cells for the treatment of age-related macular degeneration and other disorders. Induced pluripotent stem cells were initially generated in 2006 by the Yamanaka group and the technology has had dramatic implications both from an ethical and scientific standpoint [169]. The technology is a significant advancement over prior technology, as it allows researchers to generate pluripotent cells for potential therapeutic use without the controversial use of embryos. These cells are generated by reprogramming adult somatic cells using transcriptional regulators such as SOX2, OCT3/4, and Klf4 [169, 170]. These cells are then reprogrammed with similar potential as embryonic stem cells and are capable of differentiating into three germ layer cell types (mesoderm, ectoderm, and endoderm) [169, 171, 172]. These iPS cells hold great promise for the generation of RPE and photoreceptors for cell replacement therapy and create a new paradigm as a novel reservoir. Tsang et al. have generated RPE-like cells from human iPS (Fig. 1.11). Several other groups have used iPS technology to generate photoreceptors and then transplant these cells into animal models of retinal degeneration [173–175].
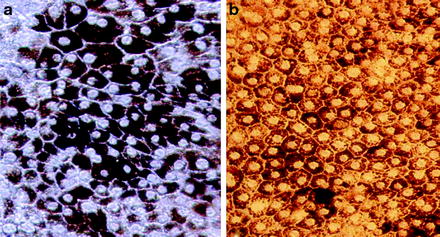
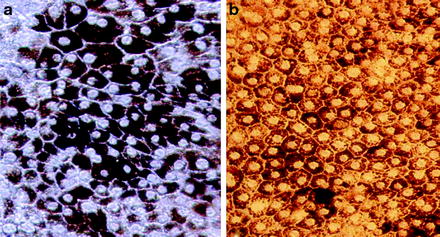
Fig. 1.11
Generation of RPE-like cells from human-induced pluripotent stem (iPS) cells. RPE derived from iPS grown on PA6 feeder cells (a, b). PA6 feeder cells exhibit stromal-derived inducing activity (SDIA), which promotes differentiation into RPE (Image courtesy of Stephen Tsang)
Induced pluripotent stem cells can also provide a platform to study disease through the use of patient-specific iPS cells. Through the generation of iPS cells from patients with specific diseases, models can be developed to express particular disease phenotypes which can then be used to understand pathophysiology of disease and determine the efficacy of therapeutic interventions [176]. These models can also be developed to help understand human inherited diseases given their clinical and genetic heterogeneity [177]. Cells derived from a particular patient can be used as a biological tool for drug discovery and toxicity testing of therapeutic agents, providing a new paradigm for personalized medicine [176].
Utilizing iPS cells as a tool for cell replacement therapy could also reduce the possibility of immune rejection given their autologous nature. Use of patient-specific iPS-derived RPE, generated from somatic cells of the potential transplant recipient with geographic atrophy, has one major and important theoretic advantage over other potential cell sources, namely, the avoidance of graft rejection. This is an important advantage, since long-term systemic immune suppression is poorly tolerated in elderly patients. Although the presence of anterior chamber-associated immune deviation (ACAID) confers some immune privilege in the subretinal space, allogeneic RPE will undergo graft rejection after subretinal transplantation unless immune suppression is used [178, 179]. Use of patient-specific iPS may circumvent graft rejection, which is one of the major challenges to ensuring graft survival in the subretinal space.
Challenges remain to the successful use of iPS cells. Induced pluripotent cells derived from the affected patient contain the predisposing mutation that caused the disease. This can provide a unique disease model but the mutation may also impede the function of the transplanted cells. These stem cells may have to first be repaired by targeted gene therapy or other techniques prior to transplantation. Additional work is also needed to translate the advances of iPS cells into clinical trials to assess safety and efficacy. Better understanding of iPS cell technology and refining the methodology of their generation will have a significant impact on retinal degenerations and regenerative medicine.
Reference
1.
Dewan A et al (2006) HTRA1 promoter polymorphism in wet age-related macular degeneration. Science 314(5801):989–992PubMed
2.
Yang Z et al (2006) A variant of the HTRA1 gene increases susceptibility to age-related macular degeneration. Science 314(5801):992–993PubMed
3.
Maller J et al (2006) Common variation in three genes, including a noncoding variant in CFH, strongly influences risk of age-related macular degeneration. Nat Genet 38(9):1055–1059PubMed
4.
DeWan A, Bracken MB, Hoh J (2007) Two genetic pathways for age-related macular degeneration. Curr Opin Genet Dev 17(3):228–233PubMed
7.
Wright AF et al (2010) Photoreceptor degeneration: genetic and mechanistic dissection of a complex trait. Nat Rev Genet 11(4):273–284PubMed
8.
Maubaret C, Hamel C (2005) Genetics of retinitis pigmentosa: metabolic classification and phenotype/genotype correlations. J Fr Ophtalmol 28(1):71–92PubMed
9.
Haim M (1992) Prevalence of retinitis pigmentosa and allied disorders in Denmark. II. Systemic involvement and age at onset. Acta Ophthalmol (Copenh) 70(4):417–426
10.
Berson EL (1993) Retinitis pigmentosa. The Friedenwald lecture. Invest Ophthalmol Vis Sci 34(5):1659–1676PubMed
11.
Phelan JK, Bok D (2000) A brief review of retinitis pigmentosa and the identified retinitis pigmentosa genes. Mol Vis 6:116–124PubMed
12.
Wang Q et al (2001) Update on the molecular genetics of retinitis pigmentosa. Ophthalmic Genet 22(3):133–154PubMed
13.
Berson EL et al (1993) A randomized trial of vitamin A and vitamin E supplementation for retinitis pigmentosa. Arch Ophthalmol 111(6):761–772PubMed
14.
Moldow B et al (1999) Effects of acetazolamide on passive and active transport of fluorescein across the normal BRB. Invest Ophthalmol Vis Sci 40(8):1770–1775PubMed
15.
Daiger SP, Bowne SJ, Sullivan LS (2007) Perspective on genes and mutations causing retinitis pigmentosa. Arch Ophthalmol 125(2):151–158PubMed
16.
Fishman GA et al (1981) Color vision defects in retinitis pigmentosa. Ann Ophthalmol 13(5):609–618PubMed
17.
Wang DY et al (2005) Gene mutations in retinitis pigmentosa and their clinical implications. Clin Chim Acta 351(1–2):5–16PubMed
18.
Williams DS (2008) Usher syndrome: animal models, retinal function of Usher proteins, and prospects for gene therapy. Vision Res 48(3):433–441PubMed
19.
Fishman GA et al (2007) Natural course of visual field loss in patients with Type 2 Usher syndrome. Retina 27(5):601–608PubMed
< div class='tao-gold-member'>
Only gold members can continue reading. Log In or Register a > to continue
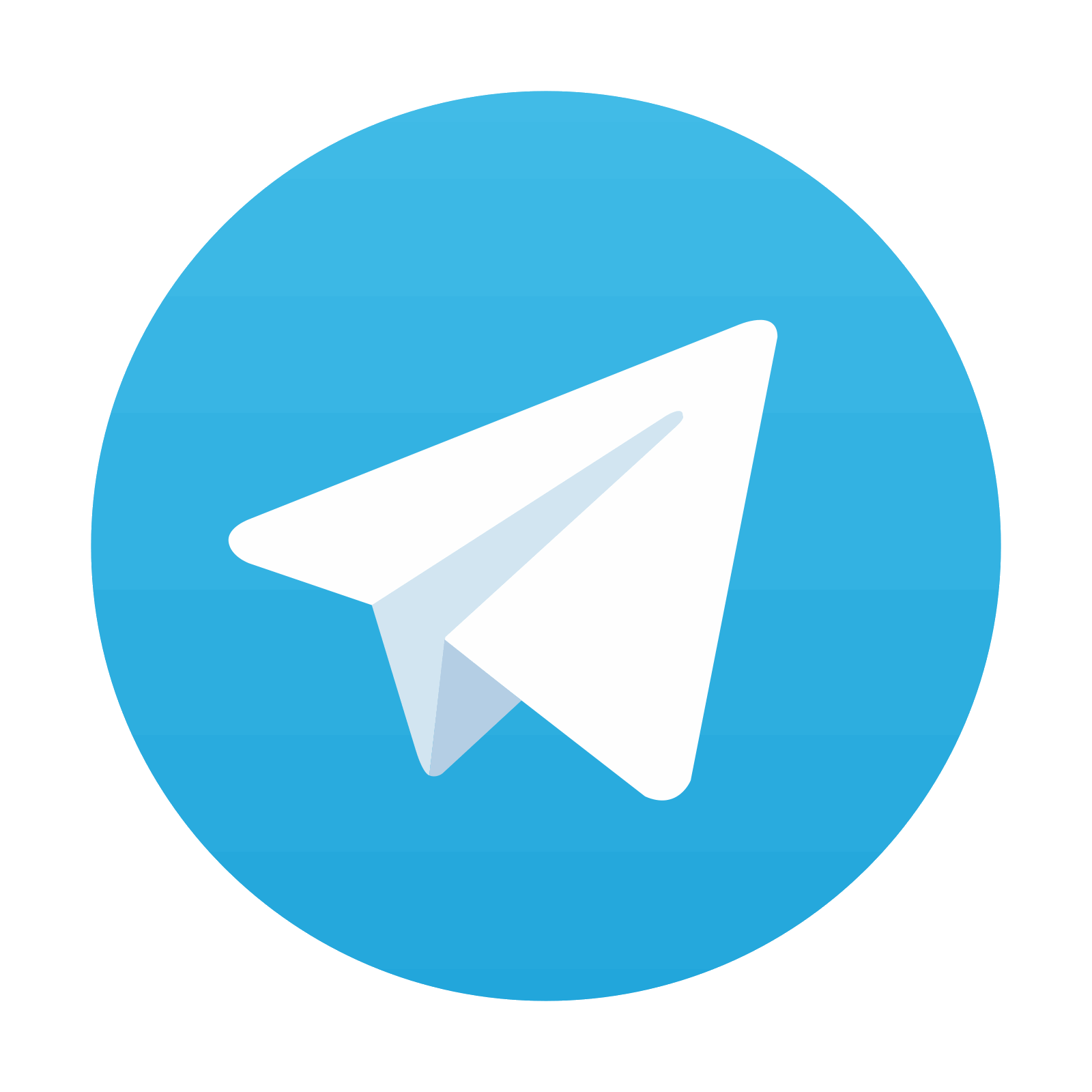
Stay updated, free articles. Join our Telegram channel
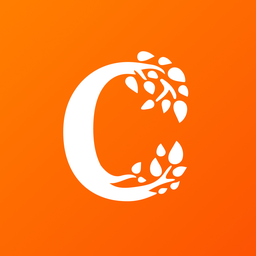
Full access? Get Clinical Tree
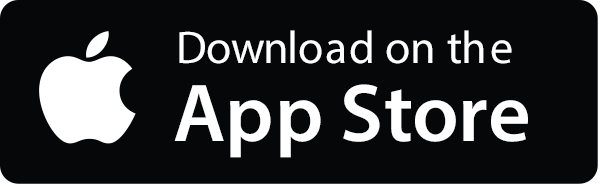
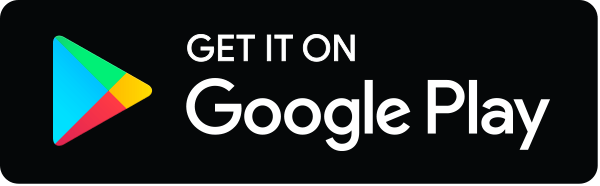