The Choroid
Alon Harris
Adam Moss
Rita Ehrlich
Introduction
The uveal tract is the vascular coat of the eye, situated between the sclera and the neuroepithelium. The uveal tract is a continuous layer composed primarily of blood vessels, connective tissue, and melanocytes, the latter of which are responsible for its distinctive brown color. It consists of the iris anteriorly, the choroid posteriorly, and the ciliary body in the middle. The iris leaflet separates the anterior compartment into anterior and posterior chambers. The ciliary body, which produces the aqueous humor, lies between the iris and the choroid. The anterior portion of the ciliary body is the pars plicata. The pars plana is the posterior portion of the ciliary body, and it merges with the choroid at the ora serrata. The choroid extends posteriorly from the ciliary body without interruption to the canal for the optic nerve. The optic canal is surrounded by the choroid and subjacent sclera.
The uvea is firmly attached to the sclera at three sites: anteriorly at the scleral spur, at the exit of the vortex veins, and posteriorly at the optic nerve. Potential spaces are present between the ciliary body and the sclera and between the choroid and the sclera. These contiguous potential spaces are called the supraciliary and suprachoroidal spaces, respectively. The entire uvea is lined by two continuous layers derived from the neuroectoderm. The layers are described in segments as the anterior and posterior pigmented epithelium of the iris; the inner nonpigmented and outer pigmented ciliary body epithelium; and the inner neurosensory retina and the subjacent retinal pigment epithelium (RPE) (Fig. 22.1).
The choroid is composed predominantly of blood vessels surrounded by melanocytes, nerves, connective tissue, and watery mucinous extracellular fluid. The choroid can be subdivided into three distinct parts from internal to external: Bruch’s membrane, the vascular layers, and the suprachoroid (Fig. 22.2). The inner boundary of the choroid is formed by Bruch’s membrane, a thin layer derived in part from the RPE and the choriocapillaris. The choroid measures 0.22 to 0.30 mm in thickness in the posterior pole and 0.10 to 0.15 mm anteriorly and peripherally. This variation in diameter is due to a greater concentration of arteries and large- and medium-sized choroidal veins in the posterior choroid. The vascular layer of the choroid is composed of three relatively distinct layers of vessels with increasing luminal diameters from internal to external: the innermost layer of capillaries (the choriocapillaris), the middle layer of medium-sized vessels (Sattler’s layer), and the outer layer of large vessels (Haller’s layer) (Fig. 22.3). These three layers are most evident posteriorly.
The uvea is supplied anteriorly by the long posterior ciliary arteries (LPCA) and the anterior ciliary arteries (ACA). Posteriorly, the choroid is supplied by the posterior ciliary arteries (PCA) and their smaller branches. The choroidal vasculature can be visualized in albino patients because of their lack of melanin pigment (Fig. 22.4). The vascular pattern may also be observed on gross examination of eyes (Fig. 22.5).
Despite being the subject of intense investigation, the exact role of the choroid remains unclear and speculative. Controversies exist regarding the angioarchitecture of the choroidal vasculature and how the vascular arrangement relates to clinical lesions demonstrated by angiography.
Embryology
The choroid develops from two embryonic tissues, the mesoderm and cranial neural crest cells. Endothelial cells of the chordoidal blood vessels are derived from mesoderm, whereas all other cells of the choroid, including stromal cells, melanocytes, and pericytes, are of neural crest origin.1,2,3 The prenatal development of the eye can be subdivided into three stages: embryogenesis, organogenesis, and differentiation. During embryogenesis, endothelial cells of the vessels originate in the paraxial mesoderm—the most medial region of the mesoderm.4 Surrounding the developing blood vessels are numerous pericytes.5 Embryogenesis continues until the end of gestational week 3, at which time the optic groove appears on either side of the midline at the cranial end of the neural folds.6
Organogenesis begins at gestational week 4 and continues through the end of week 8. During week 4, the optic vesicle forms and begins to invaginate to form the optic cup.7 Cranial neural crest cells migrate in waves to surround the optic vesicle with a layer of undifferentiated tissue. Endothelial cells begin to line spaces forming growing tubes that extend from the central axis to the caudal end of the optic vesicle. These tubes form a constantly growing and expanding plexus of primitive blood vessels. Blood flow has not yet become orderly, and the vessels are neither veins nor arteries. By the end of week 4, blood vessels cover the neural tube and extend over the external surface of the optic cup.2,8
During week 5, the hyaloid vasculature develops, forming an anastamosis with the vessels outside the optic cup.4 During week 6, the primitive choriocapillaris begins to differentiate along with the continued development of the RPE.4 Although very narrow in diameter, primitive vascular channels (Fig. 22.6) can be identified lying in close proximity to the developing RPE. Endothelial cells on the retinal and scleral sides of the capillaries remain very similar in ultrastructure at this point.9
Between weeks 5 and 7, melanin first appears in the RPE.10,11,12,13 Capillaries develop from the vascular plexus external to the pigmented areas (Fig. 22.7). These capillaries invest the optic cup; however, they are separated from the optic cup by the basement membrane of the RPE, which is the first layer of Bruch’s membrane to form.10,12,14,15,16 The formation of these capillaries is linked to pigmentation. Subjacent choroid and sclera never properly form if the RPE fails to develop, as is the situation with colobomas. Zhao and Overbeek17 used a mouse model to demonstrate that the choroid fails to form in the absence of the RPE, illustrating the interactions between RPE and periocular mesenchyme and their inductive role in the vascular development of the choroid (Fig. 22.8).
As the embryonic fissure continues to close into week 7, the periocular mesenchyme undergoes significant development to form the choroidal vasculature.14 The choriocapillaris continues to mature and becomes well formed. Endothelial cells facing the primitive Bruch’s membrane are nonfenestrated,9 and pericytes form on the endothelial cell wall adjacent to the maturing sclera.8 By week 9, the basement membrane of the capillaries appears (Fig. 22.9) and over the next 3 weeks, collagen begins to fill the space between it and the basement membrane of the RPE (Fig. 22.10).10,11,13,15,16 The production of collagen, as well as the matrix of Bruch’s membrane, is attributed to the fibroblasts that can be found between the capillaries at this stage.
Greiner and Weidman18 documented the comparative histogenesis and timing of the development of Bruch’s membrane in several species of mammals with different lengths of gestation. Although the relative timing differed among the different species studied, the order remained the same. The basement membrane of the RPE was the first to develop, followed by the collagenous layer and then the basement membrane of the choriocapillaris. The last layer to develop was an elastic tissue layer, which divided the collagenous layer into thicker inner and thinner outer layers. This differs from the previously accepted understanding of the development of Bruch’s membrane in humans. It was previously believed that the collagenous layer is formed before the appearance of the endothelial basement membrane of the choriocapillaris.18
Also during the second month, arterial branches of the ophthalmic artery and posterior ciliary arteries pass perpendicularly into the choriocapillaris. By the end of month 2, the primitive choriocapillaris is well defined. Arteries and veins can now be differentiated, the former being narrow and two to three layers thick, whereas the latter are composed only of a wide lumen lined by a single endothelial layer.14,19 Vortex veins form from the confluence of the collecting channels of the primitive choriocapillaris.8
The third stage of prenatal development involves the differentiation of primitive organs into fully or partially active organs. This process starts at the beginning of the third month. Some capillaries of the choriocapillaris enlarge to form a second layer of choroidal vessels.8 Beginning at the posterior pole and proceeding anteriorly, a new layer of capillaries emerge along the RPE to form the definitive choriocapillaris.7,19 The endothelial cells of the mature choriocapillaris are connected via tight junctions and contain numerous diaphragm-covered fenestrations on the surface facing the prospective Bruch’s membrane. Short posterior ciliary arteries (SPCA) pierce the scleral coat, which has now grown to surround the developing choroid, and to connect posteriorly to the capillaries of the choriocapillaris. The LPCA terminate in the region of the ciliary body. Several small branches enter the developing sclera to form the primitive arterial circle of Haller and Zinn.7,8
During months 4 and 5, the vasculature of the retina and uvea continue to mature, and the circle of Haller and Zinn continues to grow and develop. Capillaries are gradually shifted outward into the choroid proper and become medium-sized veins, whereas smaller capillaries fill the area vacated by these displaced vessels. Extensions from the SPCA and venules of the choriocapillaris connect to form Sattler’s layer, a layer of medium-sized blood vessels and the middle layer of the choroid.20 These changes progress from posterior to anterior as the capillaries reach their final anatomic positions. By the end of the fifth month, all three vascular layers of the choroid can be visualized.19
During the same period, ACA form and join the iris circle along with the LPCA. Later, from the sixth to ninth months, recurrent branches from the major arterial circle also develop. The SPCA also contribute submacular branches, which extend in a fanlike pattern.19
Between weeks 24 and 27, melanin is first produced by peripapillary choroidal melanocytes. Melanization proceeds anteriorly, and is complete by birth.13 The stroma of the choroid, also of neural crest origin, is highly nucleated at birth, and nuclear density increases with continued growth of the globe.11,14
Choroidal Anatomy
Bruch’s Membrane
Bruch’s membrane is a thin, acellular, well-delineated zone between the retina and choroid extending from the optic nerve to the ora serrata. Bruch’s membrane is a connective tissue layer that lies between the metabolically active RPE cells and the choriocapillaris (Fig. 22.11). Composed of elements from both the retina and the choroid, Bruch’s membrane is an integral part of the choroid. It provides a support element and an attachment site for the RPE cells. It supplies nutrition to RPE cells21 and provides a semipermeable filtration barrier that regulates ionic and metabolic exchange between the RPE and choriocapillaris. Nutrients pass from the choricapillaris to the RPE and photoreceptors, and cellular breakdown products travel in the opposite direction.22
From internal to external, Bruch’s membrane is formed of five layers: the basement membrane of the RPE, the inner collagenous zone, the elastic tissue layer, the outer collagenous zone, and the basement membrane elaborated by the endothelial cells of the choriocapillaris (Fig. 22.12). Bruch’s membrane is thickest near the optic disc, measuring 2 to 4 μm, and gradually decreases in thickness peripherally where it measures 1 to 2 μm.23 The innermost layer, the basement membrane of the RPE, is a continuous membrane measuring 0.3 μm in thickness. The outer layer, the basement membrane of the choriocapillaris, is 0.14 μm thick and is discontinuous at the intercapillary septa. The inner and outer collagenous layers are continuous and measure 1.5 and 0.14 μm, respectively. The middle elastic tissue layer is discontinuous. Normally, in a healthy globe, the layers of Bruch’s membrane are so closely interwoven that they appear continuous and cannot be separated.22,24,25,26,27
With age, drusen can accumulate on either side of the elastic layer of Bruch’s membrane.28 The two classifications of drusen, depending on where they are located, are basal linear and basal laminar deposits. Basal linear deposits accumulate between the basal lamina of the RPE and the inner collagenous zone of Bruch’s membrane (Fig. 22.13). Basal laminar deposits are found between the basement membrane and plasma membrane of the RPE, and they consist of basement membrane proteins and collagen.21
![]() Figure 22.13. Solitary nodular drusen (arrow) resting on inner surface of Bruch’s membrane. (Hematoxylin-eosin [H&E] stain, × 63) |
A morphometric study of Bruch’s membrane, the choriocapillaris, and the choroid in aging was performed by Ramrattan et al.29 in eyes obtained from patients ranging in age from 6 to 100 years with normal maculae. With increased age, the thickness of Bruch’s membrane increases by 135% from 2 μm in the first decade of life to 4.7 μm in the tenth decade.29 The capillary density of the choriocapillaris was determined as the ratio of the sum of the lengths of the lumina of the capillaries to the length of the zone in which measurements were made. Both the capillary density and diameter of the choriocapillaris and the choroidal thickness generally decrease linearly with age. There is a 45% decrease in choriocapillary density in the normal macula, from 0.75 in the first decade to 0.41 in the tenth decade. In addition, there was 34% decrease in the lumen diameter of the choriocapillaris of normal human maculas from 9.8 μm in the first decade to 6.5 μm in the tenth decade, and a reduction in the thickness of the choroid from 193 μm in the first decade of life to 84 μm in the tenth decade. Statistical analysis of the morphometric data showed that the thickness of Bruch’s membrane was directly related to age alone and that no relationship exists between age-related atrophy of the choriocapillaris and changes in Bruch’s membrane thickness. The increased thickness of Bruch’s membrane involves both the elastin and collagenous layers, and results from decreased degradation and increased production of the extracellular matrix.30 The amount of noncollagen proteins, lipid deposits, heparin sulfate, laminin, and fibronectin in Bruch’s membrane also increases in with age.30
Ultrastructurally, the basement membrane of the RPE and choriocapillaris is made of fine filaments that blend with the collagen of the adjacent collagenous zones. The basement membrane of the RPE is separated from the cytoplasmic membrane of the RPE, from which it is derived, by a 100-nm radiolucent zone. The cytoplasmic membrane of the RPE cell has many infoldings, which its basement membrane usually does not follow, although it may project slightly into the outer part of some folds.31,32 The basement membrane of the RPE is continuous with the basement membranes of the pigmented epithelium of the ciliary body and anterior pigmented epithelium of the iris and, therefore, extends from the optic disc to the pupillary edge of the iris. The basement membrane of the choriocapillaris is discontinuous at the intercapillary septa.
The inner and outer collagenous zones are made up of randomly oriented collagen fibers measuring approximately 60 nm in diameter. Many are parallel to the retina, whereas others pass from the inner collagenous zone through the elastic layer into the outer collagenous zone. At the ora serrata, the inner collagenous layer thickens, displacing the elastic layer outwardly. The collagen layer contains collagen fiber types I, III, and V. Type I collagen provides tensile strength to the tissue, type III provides elastic properties, and type V provides a connection between the basement membrane and the stromal matrix.22 Type IV collagen is found in the basement membrane of the RPE and the choriocapillaries. The solubility of collagen decreases by 50% from the first to the ninth decade of life. This is thought to be caused by the crosslinking of collagen fibers. These changes can affect the permeability through the membrane and can lead to changes in the extracellular matrix. These modifications of the collagen can also influence its vulnerability to collagenolytic enzymes from the RPE and choriocapillaris, and can affect the turnover of collagen.22
The elastic layer, the middle layer of Bruch’s membrane, is a dense, irregularly interrupted band composed of interwoven elastic tissue fibers of various thicknesses. The elastic fibers are composed of long and straight rods, with a homogeneous core and dense cortex. Variably sized spaces are present between the individual elastic fibers, which provide passageways for collagen fibers from the inner collagenous zone to the outer collagenous zone and into the intercapillary septa and subcapillary zone of the choriocapillaris (Fig. 22.14). The elastic tissue and collagenous layers of Bruch’s membrane become circularly oriented around the edge of the optic nerve.
The interfiber matrix of Bruch’s membrane is composed largely by heparin sulfate, chondrontin, and dermatan sulfate. Chondrontin sulfate is thought to provide electrolytic barrier to diffusion. Heparin sulfate is more concentrated in the basement membrane of the RPE, whereas chondroitin sulfate is more concentrated in the collagenous layer and side nearest the choriocapillaris.22 In addition to the ultrastructurally defined components of Bruch’s membrane, vesicles, linear structures, and electron-dense bodies are found in the collagenous and elastic zones.25,26,31,32 During the fourth decade of life, lipoproteins begin to be deposited and appear in Bruch’s membrane. The lipoproteins vary in diameter from 60 to 80 nm up to 300 nm. Other small granules also appear in the macular Bruch’s membrane in or near the elastic layer. The inner collagenous layer is progressively filled in the next two to four decades of life, leading eventually to the formation of a lipid wall between the inner collagenous layer and the basement membrane of the RPE33 in both the macular and peripheral retina.34 The diffusion and permeability across Bruch’s membrane depend on local pH, with a pH of 5.0 equaling the isoelectric point of maximal diffusion of local salts and glucose. At the physiologic pH, the membrane is negatively charged. Changes in the components and structure of the Bruch’s membrane can influence its diffusion properties as well as the function of the RPE cells and the outer retina.22 Nerve fibers have not been found in Bruch’s membrane.31
Choroidal Vasculature
The choroid is the most vascular portion of the eye and one of the most vascular tissues in the body,8 providing a volume of blood flow that is up to 40 times greater than that of the retinal vasculature.35 In addition, it contains many wide, but flattened, capillaries with fenestrated walls. As a result, it plays an important role in the maintenance of the retina by providing metabolites to the outer retina and RPE. In some areas of the eye, such as the fovea, the choroidal circulation is the sole source of metabolic exchange. The capillary wall is permeable to proteins and, therefore, is also capable of providing the RPE with vitamin A. Further, the choroidal blood flow dissipates the heat generated by the metabolic processes initiated when photons of light are absorbed in the pigments of the RPE and choroid. Under extreme conditions in which there is a significant increase in the environmental temperature, such as in a sauna or with irradiation of the retina, choroidal blood flow may help to cool the eye.36,37 Under normal conditions, the same blood flow helps to keep the eye warm.38
The inner-most vascular layer of the choroid, the choriocapillaris, lies adjacent to Bruch’s membrane. This layer is composed of small but richly anastomotic, fenestrated capillaries that originate at the margin of the optic nerve. The capillaries of the choriocapillaris are separate and distinct from those of the optic nerve.39 Moving externally, the next layer is composed of medium-sized vessels (Sattler’s layer). The outermost layer, known as Haller’s layer, contains the largest of the choroidal arteries and veins. Only in the posterior pole can the three layers be distinctly identified as separate layers. Elsewhere, the choroid consists only of choriocapillaris and a deeper layer of larger vessels in which individual stratum are not discernible.40 The choroidal vasculature is responsible for meeting the metabolic and oxygen requirements of the RPE cells and photoreceptors. A variety of pathologies, including choroidal coloboma and age-related macular degeneration (ARMD), result from abnormalities in the choroid vasculature.
The main morphologic and anatomic features of the choroidal vasculature have been an area of study for many decades. Original techniques included intravascular casting, scanning electron microscopy, flat preparation of mounted choroid, and histologic sections. More recently, the development of new techniques has allowed the in vivo study of the vasculature of the choroid. Laser Doppler flowmetry and angiography with both fluorescein and indocyanine green have increased our knowledge of the angioarchitecture and function of the choroid.
Arterial Blood Supply
The choroid receives its arterial blood supply from branches of the ophthalmic artery (OA), the first branch of the internal carotid artery (ICA). The OA arises from the ICA after the latter turns to pierce the dura and emerges from the cavernous sinus.41 One to five PCA arise from the OA and then typically divide into multiple branches forming groups of SPCA and LPCA (Fig. 22.15).42 In addition to the PCA, several ACA also branch from the OA to supply the choroid. Although the retinal vessels also arise from the OA, the retinal and choroidal circulations are separate and distinct.
Posterior Ciliary Arteries
Despite the significant variability that has been described, the first branches of the OA are typically PCA. In studies by Hayreh,43 nasal and temporal PCA were present in 100% and 97%, respectively, of eyes studied. Each artery branches several times in the orbit while proceeding anteriorly toward the globe and giving rise to the LPCA and SPCA. Occasionally, the LPCA or SPCA arise as a direct branch of the OA.23,44 LPCAs have also been identified as branches from the ipsilateral SPCA. The nasal and temporal PCA can be duplicated in up to 30% and 20% of individuals, respectively. Additionally, a third, superior trunk can be found in approximately 9% of individuals.8,43
The PCA play a significant role in the blood supply to both the choroid and optic nerve head. Significant interindividual variation, as well as variation between the eyes of a single individual, exists in the territories supplied by the nasal and temporal PCA in humans.45 For example, the nasal PCA may supply the entire nasal portion of the choroid and extend laterally as far as the fovea, including in its territory the optic nerve head. On the contrary, the nasal PCA may cease medial to the nasal peripapillary choroid, providing no blood supply to the optic nerve head. A watershed area exists between the supply of the medial and lateral PCA, usually as a vertically oriented zone between the optic nerve and macula. The watershed zone can be located anywhere between the fovea and the nasal border of the optic disc (Fig. 22.16).46 The position of this zone is clinically significant, because it determines the extent of the involvement of the optic nerve head should an acute ischemic insult occur as a result of the occlusion of one of the two main PCA.
Short and Long Posterior Ciliary Arteries
Each main PCA divides into one LPCA and approximately seven to ten SPCA as it reaches and penetrates the sclera at the horizontal meridian of the globe (Figs. 22.17 and 22.18). The vessels tend to cluster 2 to 2.5 mm from the dural sheath of the optic disc (Fig. 22.19), with most being found inferotemporal to the scleral entrance of the temporal LPCA.23 Some SPCA travel through the sclera, without branching, directly to the choroid. Others branch within the scleral space, providing branches to both the choroid and anterior optic nerve head. The distal branches radiate toward the equator of the globe within the outermost layer of the choroid to supply a wedge-shaped territory. On occasion, the SPCA form an extrascleral anastomosis around the optic nerve on the posterior aspect of the globe. This rich plexus receives arterial tributaries from the vessels on the dural sheath of the optic nerve, arteries accompanying the extraocular muscles, short ciliary nerves, LPCA, SPCA, and small vessels from the surrounding connective tissue.47 The SPCA supply most of the anterior optic nerve head and the posterior portion of the choriocapillaris.48
![]() Figure 22.18. Gross specimen of posterior of globe shows many of the structures shown in Figure 22.17: vortex veins (v), optic nerve (on), muscular tendon of inferior oblique (io), tendon of superior oblique (so), short posterior ciliary arteries (arrows), short posterior ciliary nerves (n), and long posterior ciliary artery and nerve (arrowhead). |
The nasal and temporal paraoptic SPCA form an elliptical circle around the optic nerve posterior to the suprachoroidal space, about 400 μm from the optic nerve head margin.49 This circle, named the arterial circle of Haller and Zinn, transmits recurrent choroidal branches, as well as pial branches and penetrating branches to the optic nerve.50,51 This anastomosis and its branches provide a significant proportion of blood to the optic nerve head. The diameter of the vessels of the circle range between 20 and 230 μm, with an average of 123 μm.49
Most SPCAs are termed distal SPCA, which enter the sclera a distance away from the optic nerve head and run radially toward the equator. Each distal SPCA supplies a sector of the choroid extending from the posterior pole to the equator.52 Approximately 30% of normal eyes also have a cilioretinal artery that supplies the retina, which is often a branch of an SPCA.53 Both distal and paraoptic SPCA supply wedge-shaped areas of choroid. Radiating outward from the macula, potential watershed zones exist between the temporal SPCA. As documented by electron microscopy and casting studies, choroidal capillaries are flattened, which provides a larger surface area for metabolic exchange with the overlying RPE.50
The anterior choriocapillaris is supplied by the two LPCA and the ACA. The LPCA can arise as direct branches of the OA, branches from the respective PCA, or as branches of an SPCA. Running adjacent to the long posterior ciliary nerve, the LPCA pierce the sclera 3 to 4 mm from the optic nerve and outside the ring of the short ciliary nerves.23 After piercing the fibrous sclera, the nasal and temporal LPCA course anteriorly and branch near the ora serrata, each contributing three to five branches posteriorly to supply the choriocapillaris anterior to the equator. The LPCA continues radially in the horizontal meridian forward to supply the iris and ciliary body.42,54 On the temporal side, the LPCA supplies a sector of the choroid temporal to the macula (Figs. 22.20 and 22.21).
Anterior Ciliary Arteries
The ACA course anteriorly along the rectus muscles and pass through the sclera. Two ACA are associated with each superior, inferior, and medial rectus muscle; only one ACA follows the path of the lateral rectus.55 All ACA are direct branches of the OA, except for one accompanying the lateral rectus muscle, which is typically a branch of the lacrimal artery. The ACA then travel through the supraciliary space before entering the ciliary muscle and terminating at the major arterial circle of the iris. This arterial plexus encircles the anterior uvea, providing 10 to 12 large recurrent tributaries to the anterior choriocapillaris, which anastomose with branches of the SPCA in the choroid.23,39,44,55 A potential watershed zone situated in the equatorial region exists between the PCA and ACA.46
Historically, the anatomy of the PCA and their branches was evaluated by postmortem cast studies. Microvascular casting is a procedure in which neoprene latex and methyl methacrylate are injected into vessels under high pressures. These postmortem studies in both animals and humans showed a lack of segmental distribution that was attributed to the ability of the vessels to anastomose freely with each other and with ACA. These original studies also revealed interarterial and arteriovenous anastomoses throughout the choroid, forming a freely communicating vascular bed of the choroid.56,57,58,59,60 These findings appeared to contradict the localized lesions that were observed clinically as a result of inflammatory, ischemic, degenerative, and metastatic diseases. A major limitation of microvascular casting is that the usage of such high pressures has potential to create vascular conduits that are otherwise absent or nonfunctional in vivo.
As a result, there has been significant debate about the physiology of the choroidal vasculature. This discrepancy prompted investigators such as Hayreh52,54 to use in vivo models to evaluate the vascular patterns of the PCA. Experimental occlusion of the SPCA and LPCA in Rhesus monkeys resulted in a localized choroidal filling defect in a segmental pattern corresponding to the occluded artery, without compensatory filling from the adjacent vessels. Similar findings have been reported more recently in the peripapillary choroid.61 Numerous other investigators have also conducted in vivo studies to document segmental and end-arterial vascular systems.62,63,64 As technology continues to progress and newer technologies become available for the study of the choroidal vasculature, this continues to be an area of debate.
Choriocapillaris
The choriocapillaris is a single layer of broad capillaries lying in a plane neighbored externally by the vessels of the choroid and internally by Bruch’s membrane. The anterior choriocapillaris is supplied by recurrent branches of the LPCA and ACA, whereas the posterior choriocapillaris is supplied by SPCA. The choriocapillaris lacks functional anastomoses between its anterior and posterior circulation, creating a peripheral choroidal watershed zone.39 The choriocapillaris supplies oxygen and nutrients to Bruch’s membrane and the outer third of the retina, except for the macula. Arterioles and venules join the choriocapillaris on its external surface at a perpendicular or oblique angle, or from within the same plane. The diameter of the capillaries in the choriocapillaris ranges from 20 to 50 μm. The choriocapillaris is thickest and has the greatest density of capillaries in its posterior region.65 This leads to regional differences in flow patterns of the choriocapillaris, providing a more highly efficient system of outflow in the posterior regions than in the peripheral zones.66
Controversy exists regarding the organization of the choriocapillaris. Hayreh52,67,68 first described the choriocapillaris as a homogeneous, lobular structure (Fig. 22.22). Based on fluorescein angiograms in monkeys, it was believed that each lobule contained a centrally located feeding arteriole, and was drained by peripherally located collecting venules.52,67,68 In his preliminary angiography studies, Hayreh67 demonstrated a segmental blood-flow distribution and that arteries behaved as end-arteries. These lobules acted as independent units, and were arranged as a mosaic bordered by the venous channels. Additional investigators have similarly found that cross-flow between lobules does not normally occur.69
Using choroidal vascular casts injected with neoprene latex, investigators differentiated between precapillary arterioles and venules by observing vascular filling.70 In the peripheral choroid, a particular pattern was identified in which a precapillary arteriole supplied an area 1 to 2 mm in diameter. Venous drainage divided the contiguous circular regions. Thus, it was thought that arterioles behaved as end-arteries despite being interconnected. No such organization was observed elsewhere in the choroid; in the posterior and equatorial choroid, many arterioles were interconnected via the choriocapillaris.
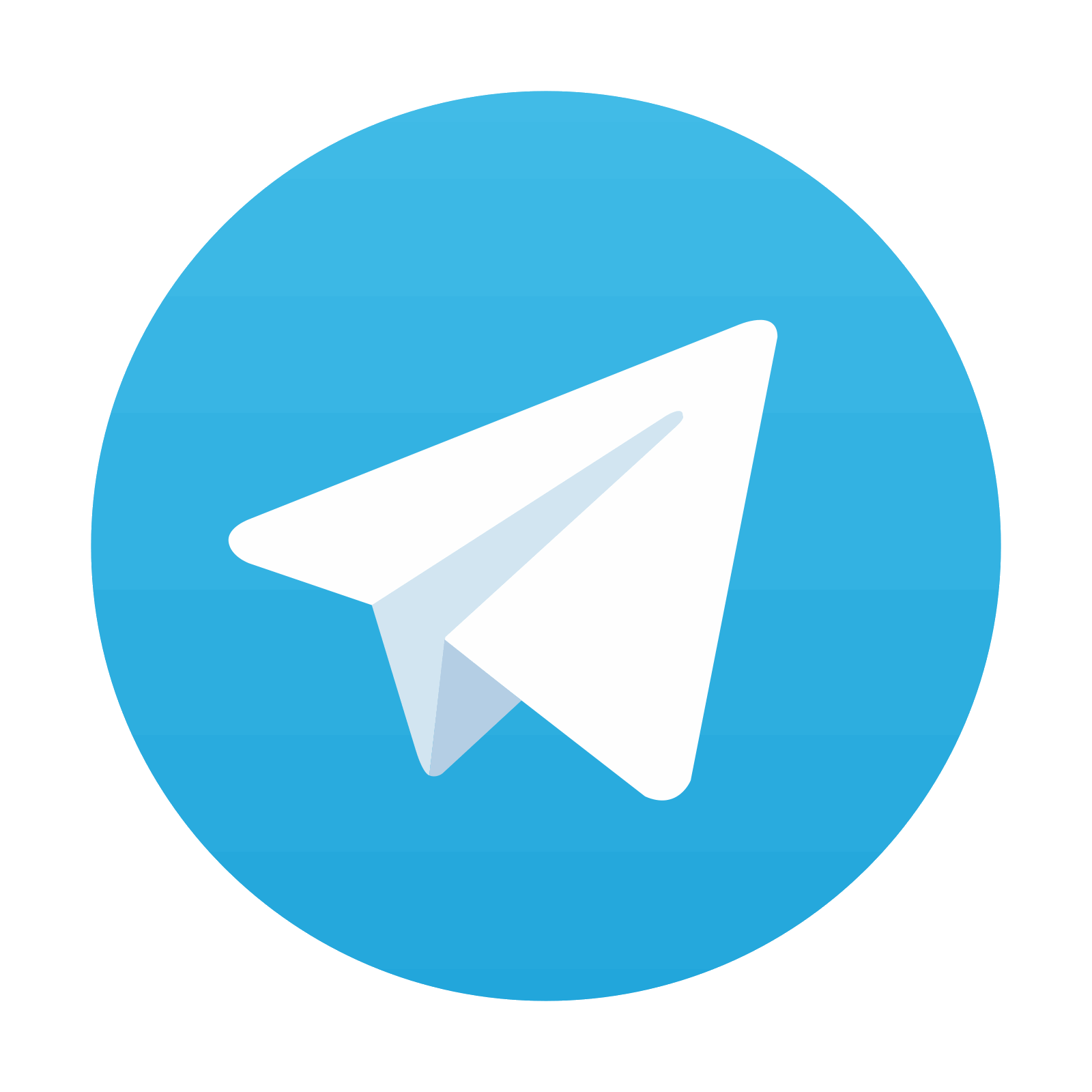
Stay updated, free articles. Join our Telegram channel
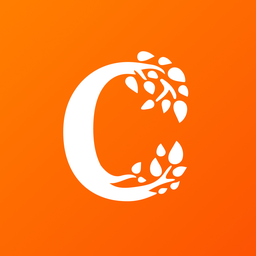
Full access? Get Clinical Tree
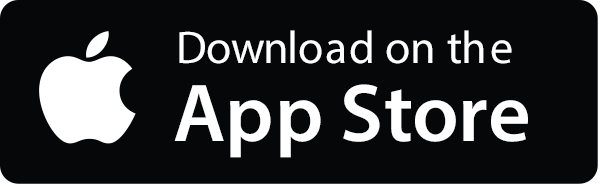
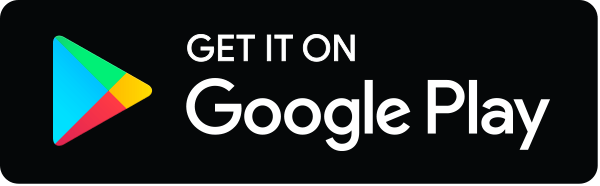