Surgical Anatomy of Vitreous and the Vitreoretinal Interface
J. Sebag
Historical Perspective
According to Duke-Elder,1 the first descriptions of vitreous structure proposed that vitreous is composed of “loose and delicate filaments surrounded by fluid.” During the 18th and 19th centuries, there prevailed no fewer than four major theories of vitreous structure: the “alveolar theory,”2 the “lamellar theory,”3 the “radial sector theory,”4 and the “fibrillar theory.”5,6 The elegant studies of Szent-Gyorgi7 supported the “fibrillar” descriptions of Retzius6 and introduced the concept that vitreous structure changes with age. The work of Baurmann,8 Stroemberg,9 and Redslob10 showed that many of the early studies were flawed by artifacts resulting from the use of tissue fixatives. Thus, it was anticipated that the use of slit lamp biomicroscopy to study vitreous structure would eliminate this problem, because investigations could be performed either in vivo or with fresh specimens in vitro avoiding artifacts. Yet, the use of in vivo slit lamp biomicroscopy spawned an equally varied set of descriptions: Gullstrand11 saw membranes, Koeppe12 described vertical and horizontal fibers, and Baurmann13 saw a grill-like pattern of darker and lighter bands resembling several layers of chain-linked fences. Even the use of dark-field microscopy in vitro resulted in various interpretations, ranging from Goedbloed’s14 description of fibrillar structures to Friedenwald and Stiehler’s15 description of concentric sheets and Eisner’s16 observation of “membranelles.” In Eisner’s studies, dissected human vitreous was found to contain membranous structures that coursed from the region about the lens in a circumferential pattern, parallel with the vitreous cortex, to insert at the posterior pole. Eisner has described these “membranelles” as funnels that are packed into one another and diverge outward and anteriorly from the prepapillary vitreous. He named these membranelles “tractae” according to their location. Worst17 has also studied dissected human vitreous and claimed that the “tracts” of Eisner constitute the walls of “cisterns” within the vitreous. In Worst’s studies, these cisterns are visualized by filling with white India ink. He has studied the premacular vitreous in great detail and has proposed the existence of a “bursa premacularis,” which he described as a pear-shaped space that is connected to the cisternal system in front of the ciliary body. In a more recent study, a different aqueous dye (fluorescein) was used to demonstrate this same premacular region of liquefied vitreous.18
Vitreous Examination Techniques
Beginning with the introduction of the ophthalmoscope by Helmholz, examiners of the eye have long benefited from advancing technology and the development of ever more powerful examination tools. Indeed, today both morphologic and functional and physiologic aspects of the eye can be evaluated with the aid of lasers.20 Of all the different parts of the eye, evaluation of vitreous, however, has advanced least in terms of the development of accurate and reproducible clinical examination techniques. This is evident in the multitude of descriptions of vitreous structure that resulted after the introduction of slit lamp biomicroscopy and the various different interpretations that exist to this day.
This controversy stems from the fact that vitreous examination essentially consists of visualizing a structure intended to be virtually invisible.21,22 The Tyndall phenomenon forms the basis of vitreous examination. This can be defined as the scattering of incident light by opaque structures or particles in an otherwise transparent medium. Essential to the success of achieving an adequate Tyndall effect clinically is maximizing pupil dilation in the patient and dark adaptation in the examiner.23 Because the Tyndall effect increases with an increasingly large subtended angle between the axis of illumination and the line of observation (up to a maximum of 90 degrees), a widely dilated pupil enhances this effect. Some observers24 propose that green light further enhances the Tyndall effect. This concept has been incorporated in a biomicroscope that utilizes a green laser to enhance visualization of the vitreoretinal interface.25
Before examining the individual parts of vitreous, it is useful to consider the overall shape of the entire vitreous as a clue to characterizing vitreoretinal pathology. Charles26 has described a “problem-oriented” approach to preoperative vitreous examination in which an appreciation of the configuration and mobility of the entire vitreous can guide the operative approach. For example, the identification of a “cone” in a partial posterior vitreous detachment (PVD) and the characterization of the locations and number of apices in this cone can help in the accurate diagnosis of vitreoretinal pathology and determine the correct surgical approach.
Anterior Vitreous
Examination of the anterior vitreous can be done at the slit lamp immediately after examination of the anterior segment and does not require gel solutions or a contact or preset lens, which are used to examine the posterior vitreous. In the absence of a crystalline or implanted intraocular lens, vitreous prolapse into the anterior chamber may be an important finding in terms of vitreocorneal touch and the risks of corneal endothelial cell dysfunction.27 Vitreous adhesions to a cataract wound or to the iris may be important in the pathogenesis of postoperative cystoid macular edema. Focal opacities in the anterior vitreous can be seen at the slit lamp, providing important clues as to the possible presence of posterior pathology. For example, particulate opacities in vitreous can be the earliest pathologic change in retinitis pigmentosa and, as such, would provide an important aid to diagnosis.23 Nonpigmented cells can be indicative of inflammation at the vitreous base or infections in the retina. Pigmented cells should raise the examiner’s index of suspicion for peripheral retinal tears or detachment. Lacqua and Machemer,28 as well as Scott,29 have described that an increase in the number and size of pigmented cells in vitreous of patients with retinal detachment (preoperatively or postoperatively) heralds the development of proliferative vitreoretinopathy (PVR). Bleeding can be associated with red blood cells in the anterior vitreous. Various neoplastic diseases, such as endophytic retinoblastoma, choroidal melanoma, and reticulum cell sarcoma, can also result in anterior vitreous cells.
Anterior vitreous structures, such as Mittendorf’s dot, a remnant of embryonic hyaloid vessel regression, can be seen at the slit lamp and should alert the examiner to the possibility of other developmental disorders, such as persistent hyperplastic primary vitreous in the fellow eye.30
Central and Posterior Vitreous
Examination of the central and posterior vitreous can rarely be achieved without the use of either a preset or contact lens. The advantage offered by this form of posterior vitreous biomicroscopy is that the avoidance of a contact lens facilitates the “ascension/descension” gaze technique whereby eye movements are used to displace the vitreous body.31 It is principally this feature that makes the preset approach superior to contact lens systems for examination of the posterior vitreous.32 Preset lens biomicroscopy can be performed either with a plano concave lens (e.g., -55 to -58.6 diopter [D] lenses of Hruby) or various convex lenses (e.g., +32 D, +58.6 D, or +60 D). The plano concave lenses produce a highly magnified, narrow-field, erect image with visualization of the posterior pole, although it is difficult to achieve an adequate illumination-observation angle. Peripheral examination can be performed only by varying the position of the fixation point of the eye, and the quality of the image is reduced by optical distortions. Convex lenses were first proposed in 195333 and involve the use of a +55 D or higher preset lens with at least a 10 degree illumination-observation angle. The resultant image is inverted and can be photographed34 (Fig. 1). Preset lens biomicroscopy during ocular saccades can be helpful in visualizing structures such as an operculum or the prepapillary ring (Weiss’s) in the posterior vitreous cortex that may have descended inferiorly in the presence of a PVD with vitreous syneresis (collapse). Indeed, it is now common practice to use a +78 D or +90 D biconcave handheld lens that at the slit lamp provides a stereoscopic, inverted image of high quality that also enables the performance of the ascension/descension examination technique.
Peripheral Vitreous
The major difficulty in examining the peripheral vitreous results from a loss of stereopsis.31 When examining the periphery, the circular pupil becomes an elliptic aperture, making it difficult to obtain an adequate view with both of the observer’s eyes. This is more of a problem in the horizontal than vertical meridians, because the examiner’s two eyes are positioned horizontally. Schepens31 suggested reducing the illumination-observation angle, rotating the slit beam to the axis of the meridian being observed, positioning both arms of the biomicroscope opposite the meridian under observation, and reducing the interpupillary distance of the slit lamp eyepieces as ways of minimizing the loss of stereopsis.
A contact lens is often required for adequate visualization of the periphery. Traditionally, the Goldmann 3-mirror lens has been most popular as a tool for peripheral vitreous and retinal examination.35 Jaffe36 has described a plan for vitreous examination with a contact lens beginning with the central region, followed by establishing the presence or absence of a PVD and identifying the extent of detachment by following the posterior vitreous cortex peripherally. He recommended using a widened slit lamp beam and “rocking” the joystick to enhance visualization of the posterior cortex. Schepens31 described this maneuver as the “oscillation technique” in which the position of the slit lamp beam is moved side to side (for a vertical slit) or up and down (for a horizontal slit). The two positions of the beam are altered so as to alternate between direct and retro illumination of a structure or point of interest. Retro-illumination is especially useful for identification of particulate or cellular opacities in the posterior vitreous. Schepens31 further describes the use of a tilted slit lamp column to enhance visualization of the peripheral vitreous. Eisner16 has devised a cone-shaped apparatus that fits onto a Goldmann 3-mirror lens and enables peripheral scleral indentation during slit lamp biomicroscopy with a contact lens. This can facilitate visualization of the peripheral retina, ora serrata, and posterior pars plana ciliaris.
The recent development of inverted-image contact lenses with various-sized fields has greatly enhanced stereoscopic examination of the fundus and vitreous and they are now routinely used for laser photocoagulation therapy of the fundus. The Mainster Retina Laser Lens is a +61 D convex aspheric contact lens that produces a real inverted image of about 45 degrees of the posterior fundus with excellent stereopsis.37 The “panfunduscopic” lens is a +85 D convex spheric lens that provides less magnification and image clarity but has a wider angle view and can be useful for peripheral examination to 60 or 70 degrees with a 15-degree tilt.
Opacified Vitreous
When opacification of the ocular media makes examination of vitreous difficult, worthwhile information can be garnered from careful study, nevertheless. As pointed out by Charles,26 much can be learned from studying the geometric configuration of a partly opaque or opacified vitreous. When opacification is advanced, however, real-time ultrasonography can be helpful in defining the consistency of the opacification, the three-dimensional configuration of the opaque vitreous, mobility, and the presence or absence of structural pathology behind the corpus vitreous. Fischer et al.38 demonstrated an excellent agreement existed between kinetic ultrasonography and conventional clinical examination techniques in subjects with clear ocular media. Arzabe et al.39 had similar findings in patients with proliferative diabetic retinopathy.
Future Examination Techniques
Although the aforementioned methods have been useful to date, the future will see a shift from our present reliance on structural changes at the cellular and tissue level, to the ability to detect physiologic and molecular abnormalities.40 Even Optical Coherence Tomography,41 the most recent innovation in posterior segment imaging,22 does not provide much more than cellular or structural information. Techniques such as Raman spectroscopy42 and dynamic light scattering43,44 are being developed to provide insights on a molecular level that will afford earlier disease detection and enable therapy to prevent progression to the cellular and tissue changes which are currently recognized as disease.
Molecular Structure
Although vitreous is 98% water, this tissue has a gel-like consistency with viscoelasticity. These properties result from hyaluronan and collagen, which are the major structural components of vitreous. The molecular composition and supramolecular organization of vitreous45,46,47 are very important considerations, as future surgical techniques will incorporate adjunctive therapy with drugs, known as “Pharmacologic Vitreolysis” 48,49,50,51 to facilitate and improve surgery, especially at the vitreo–retinal interface.
Hyaluronan
Hyaluronan (HA) is a major macromolecule of vitreous. Although it is present throughout the body, HA was first isolated from bovine vitreous in 1934 by Meyer and Palmer. HA is a long, unbranched polymer of a repeating disaccharide (glucuronic acid β (1,3)-N-acetylglucosamine) moieties linked by β 1–4 bonds.52 It is a linear, left-handed, three-fold helix with a rise per disaccharide on the helix axis of 0.98nm.53 The sodium salt of HA has a molecular weight of 3 to 4.5 × 106 in normal human vitreous.54 HA is not normally a free polymer in vivo, but is covalently linked to a protein core, the ensemble called a proteoglycan.
Collagen
Recent studies46 of pepsinized forms of collagen confirmed that vitreous contains collagen type II, a hybrid of types V/XI, and type IX collagen in a molar ratio of 75:10:15, respectively. In the entire body, only cartilage has a similarly high proportion of type II collagen as vitreous, explaining why certain inborn errors of type II collagen metabolism affect vitreous as well as joints. Vitreous collagens are organized into fibrils with type V/XI residing in the core, type II collagen surrounding the core, and type IX collagen on the surface of the fibril. The fibrils are 7 to 28 nm in diameter,55 but their length in situ is unknown.
Supramolecular Organization
As originally proposed by Balazs136 and more recently described with precision by Mayne56, vitreous is a dilute meshwork of collagen fibrils interspersed with extensive arrays of HA molecules. The collagen fibrils provide a scaffold-like structure that is ‘inflated’ by the hydrophilic HA. If collagen is removed, the remaining HA forms a viscous solution; if HA is removed, the gel shrinks but is not destroyed. Comper and Laurent57 proposed that electrostatic binding occurs between the negatively charged HA and the positively charged collagen in vitreous.
Bishop46 has proposed that to appreciate how vitreous gel is organized and stabilized requires an understanding of what prevents collagen fibrils from aggregating and by what means the collagen fibrils are connected to maintain a stable gel structure. Studies46 have shown that the chondroitin sulfate chains of type IX collagen bridge between adjacent collagen fibrils in a ladderlike configuration spacing them apart. Such spacing is necessary for vitreous transparency, because keeping vitreous collagen fibrils separated by at least one wavelength of incident light minimizes light scattering, allowing the unhindered transmission of light to the retina for photoreception. Bishop proposed that the leucine-rich repeat protein opticin is the predominant structural protein responsible for short-range spacing of collagen fibrils. Concerning long-range spacing, Scott et al.58 and Mayne et al.59 have claimed that HA plays a pivotal role in stabilizing the vitreous gel.
The topographic heterogeneity in the distribution and density of both collagen and proteoglycans (especially HA) molecules creates a heterogeneous molecular network. This inhomogeneity renders an optical anisotropy to vitreous that has been measured using laser light-scattering techniques.60 Dynamic light scattering may provide a useful noninvasive technique to measure the molecular44 physicochemical50,51 properties of vitreous.
Vitreous Body
The fully developed, adult form of the “secondary vitreous” is known as the vitreous body. In an emmetropic adult human eye it is approximately 16.5 mm in axial length, with a depression anteriorly just behind the lens (patellar fossa). Various structures and regions within the vitreous body are named after anatomists and histologists (Fig. 2). The hyaloideocapsular ligament (of Weiger) is the annular region 1 to 2 mm in width and 8 to 9 mm in diameter where the corpus vitreous is attached to the posterior aspect of the lens. “Erggelet’s” or “Berger’s” space is at the center of the hyaloideocapsular ligament. Arising from this space and coursing posteriorly through the central vitreous is the canal of Cloquet (Figs. 2, 3H, 4), which is the former site of the hyaloid artery in the primary vitreous. The former lumen of the artery is an area devoid of vitreous collagen fibrils, surrounded by multifenestrated sheaths that were previously the basal laminae of the hyaloid artery wall.61,62 Posteriorly, Cloquet’s canal opens into a funnel-shaped region anterior to the optic disc known as the area Martegiani.
![]() Figure 2. Schematic diagram of vitreous anatomy. (Schepens CL, Neetens AE [eds]: The Vitreous and Vitreoretinal Interface, New York: Springer-Verlag, 1987) |
Investigations of vitreous structure have long been hampered by transparency within the vitreous body and the absence of easily recognized landmarks. Without landmarks, removal of the vitreous from the eye results in a loss of orientation. The transparency of vitreous renders observation in conventional diffuse light unrewarding. Attempts to study vitreous structure with opaque dyes do visualize the areas filled by dye but obscure the appearance of adjacent structures. The use of the contrast-enhancing techniques of histology usually involves tissue fixation, which includes dehydration. Because vitreous is 98% water, dehydration induces profound alteration of internal morphology. Consequently, any investigation of vitreous structure must overcome these difficulties.
Dissection of the outer layers of the eye (sclera, choroid, and retina) can be performed and the “naked” vitreous body can be maintained intact and attached to the anterior segment of the eye (Fig. 5). This enables study of internal vitreous morphology without a loss of intraocular orientation. Depending on the age of the subject and consequently the degree of vitreous liquefaction,63 the dissected vitreous will remain solid and intact (young individuals, see Fig. 5) or will be flaccid and collapse. The latter is most often the case in specimens from older adults, and consequently vitreous turgescence must be maintained to avoid distortion of intravitreal morphology. Immersion of a dissected vitreous specimen that is still attached to the anterior segment into a physiologic solution maintains vitreous turgescence and avoids structural distortion.
The limitations induced by vitreous transparency were first overcome by Goedbloed14 and then by Friedenwald and Stiehler15 and by Eisner,16 who employed dark-field slit illumination of the vitreous body to achieve visualization of intravitreal morphology. Illumination with a slit lamp beam directed into the vitreous from the side and visualization of the illuminated portion from above produces an optical horizontal section (Fig. 6). The thickness of this cross section can be varied, as can the vertical position (level) of the cross section. This enables visualization of any portion of the vitreous that is of interest. The illumination-observation angle of 90 degrees that is achieved using this technique maximizes the Tyndall effect and thus overcomes the limitations induced by vitreous transparency. Furthermore, the avoidance of any tissue fixation, contrast solutions, or dyes eliminates the introduction of many of the artifacts that flawed earlier investigations.
![]() Figure 6. Schematic diagram of dark-field slit illumination system for the study of vitreous structure. The dissected specimen is mounted and immersed in a Lucite chamber containing a physiologic solution (see Fig. 2B). A slit lamp beam is shown from the side through the intact vitreous. The beam enters and exits laterally, avoiding any scattering of light by the structures of the anterior segment. The position of the beam can be raised or lowered, and the thickness of the illuminated portion can be regulated. Observation is performed from above, perpendicular to the plane of illumination. This achieves a 90° illumination/observation angle and thus maximizes the Tyndall effect. (Sebag J: The Vitreous—Structure, Function and Pathobiology, New York: Springer-Verlag, 1989) |
Researchers64,65,66,67 have used these techniques to identify that, within the adult human vitreous, there are fine, parallel fibers coursing in an antero-posterior direction, as shown in Figures 3 B, 3 C, Figure 7 and Figure 8. The fibers attach into the vitreous base (Figs. 3 H and 7) where they splay out anterior and posterior to the ora serrata. As the peripheral fibers course posteriorly, they are circumferential with the vitreous cortex, whereas central fibers “undulate” in a configuration parallel with Cloquet’s canal.6 The fibers are continuous and do not branch. Posteriorly, these fibers attach into the vitreous cortex (Fig. 3 E, F), but not the internal limiting lamina of the retina.
Ultrastructural studies66 have demonstrated that collagen organized in bundles of packed, parallel fibrils (Fig. 9) is the only microscopic structure that could correspond to these fibers. It has been hypothesized that the visible vitreous fibers form when HA molecules no longer separate the microscopic collagen fibrils, resulting in the aggregation of collagen fibrils into bundles from which HA molecules are excluded.68,69 Eventually, the aggregates of collagen fibrils attain sufficiently large proportions that can be visualized in vitro (Figs. 3, 7, and 8) and clinically. The areas adjacent to these large fibers have a low density of collagen fibrils in association with HA molecules and, therefore, do not scatter light as intensely as the larger bundles of aggregated collagen fibrils. Furthermore, these adjacent areas offer relatively little resistance to bulk flow through vitreous, because they are largely occupied by hydrated HA.
Age-Related Changes
Changes occur in vitreous structure throughout life.68,69 Figure 4 demonstrates typical vitreous structure during late prenatal stages. Within the vitreous body, there are no structures other than the remnants of the hyaloid artery oriented toward the prepapillary region. The vitreous body is relatively small and has an overall dense appearance with marked density at the outermost “shell” corresponding to the vitreous cortex. The generalized density of the vitreous likely relates to that, at this stage of development, collagen and proteoglycan(s) other than HA are the principal structural components.70,71 HA synthesis begins after birth, increasing transparency by the aforementioned mechanisms.
During childhood, only the vitreous cortex scatters incident light and thus appears dense by dark-field slit microscopy (Fig. 10). No fibers are visible within the vitreous until middle age (Figs. 3, 7, and 8). During old age, these fibers become thickened and tortuous, associated with many pockets of liquid vitreous and a collapsed (syneretic) appearance (Fig. 11). These changes are the result of age-related biochemical alterations in the composition and organization of the molecular components that simultaneously result in vitreous liquefaction and fiber formation. Pockets of liquid vitreous have classically been called “lacunae.” In addition to having a low density of collagen during youth, the central vitreous is the first region to undergo liquefaction during middle age.16 A report by Kishi and Shimizu18 described the presence of a “posterior vitreous pocket” that the authors interpreted to represent an anatomic entity. More than 95% of the eyes examined in that study, however, were from persons aged 65 years or older. Thus, these findings represent the result of age-related vitreous liquefaction in the central precortical posterior vitreous.72 Such changes could also explain the preferential pooling of aqueous dyes such as India ink17 placed onto the anterior vitreous and allowed to collect anterior to the macula in what appears as a “bursa.”17,73,74 The use of fluorescein by Kishi and Shimizu18 represents but another way of demonstrating the phenomenon of vitreous liquefaction in this region.
In a large autopsy study of formalin-fixed human eyes, O’Malley75 provided quantitative confirmation of these observations. He found that more than half of the vitreous body was liquefied in 25% of persons aged 40 to 49 years, and that this increased to 62% of individuals aged 80 to 89 years. Oksala76 used ultrasonography in vivo to detect echoes from gel-liquid interfaces in 444 normal human eyes and observed echoes in 5% of young persons, in more than half of those aged 51 to 60 years, and in more than 80% of persons older than 60 years. Vitreous liquefaction actually begins much earlier than the ages at which clinical examination or ultrasonography detects changes. Flood and Balazs63 found evidence of liquid vitreous after the age of 4 years and observed that by the time the human eye reaches its adult size (ages 14 to 18 years) approximately 20% of the total vitreous volume consists of liquid vitreous. In these postmortem studies of fresh, unfixed human eyes it was observed that after the age of 40 years there is a steady increase in liquid vitreous that occurs concomitantly with a decrease in gel volume. By the ages of 80 to 90 years, more than half the vitreous is liquid. The finding that the central vitreous is where fibers are first observed is consistent with the observation that the central vitreous is the first area to undergo liquefaction, supporting the concept that dissolution of the HA–collagen complex results in the simultaneous formation of liquid vitreous and aggregation of collagen fibrils into bundles of parallel fibrils. A large pocket of liquid vitreous could be clinically mistaken as a PVD. At surgery, a mistaken entry could be made into a large pocket of liquid vitreous as entry into the retro-cortical space created after PVD. If there is a split in the posterior vitreous cortex (“vitreoschisis”; see below), surgical entry into the schisis cavity could likewise be misinterpreted as entry behind the posterior vitreous cortex. When the entire posterior vitreous cortex detaches from the retina, an overall reduction in the size of the vitreous body occurs, owing to the collapse (syneresis) of the vitreous that occurs when liquid vitreous enters the space behind the posterior vitreous cortex, anterior to the retina. This displacement of liquid vitreous occurs through the prepapillary “hole” and possibly the premacular or other portion of the posterior vitreous cortex and is an important event in the pathogenesis of PVD.
Vitreous Base
The vitreous base is a three-dimensional zone that extends 1.5 to 2 mm anterior to the ora serrata, 1 to 3 mm posterior to the ora serrata,77 and several millimeters into the vitreous body itself.78 The vitreous base is virtually inseparable from the peripheral retina. Thus, dissection of retina off the vitreous cortex in autopsy eyes always results in a band of retina that remains attached to the peripheral vitreous at the vitreous base (Fig. 5). Similarly, clean dissection of the vitreous base and peripheral vitreous cortex from the retina during vitrectomy surgery is extremely difficult to achieve. The ultrastructural correlate of this surgical observation may relate to vitreous fiber insertions at the vitreous base where they “splay out” (Fig. 8) to insert anterior and posterior to the ora serrata (Fig. 3 H). The anterior-most fibers form the “anterior loop” of the vitreous base (Fig. 12), a structure that is important in the pathogenesis and surgery of anterior PVR. In the posterior portion of the vitreous base, vitreous fibers are closer together than elsewhere. Gartner79 has found that in humans the diameters of collagen fibrils in the vitreous base range from 10.8 to 12.4 nm, with a major period of cross striations of 50 to 54 nm. Hogan77 demonstrated that just posterior to the ora serrata, heavy bundles of vitreous fibrils attach to the basal laminae of retinal glial cells. Studies by Gloor and Daicker80 showed that cords of vitreous collagen insert into gaps between the neuroglia of the peripheral retina. They likened this structure to Velcro and proposed that this would explain the strong vitreoretinal adhesion at this site. In the anterior vitreous base, fibrils interdigitate with a reticular complex of fibrillar basement membrane material between the crevices of the nonpigmented ciliary epithelium.81 The vitreous base also contains intact cells that are fibroblastlike anterior to the ora serrata and macrophagelike posteriorly.81 Damaged cells in different stages of involution and fragments of basal laminae, presumed to be remnants of the embryonic hyaloid vascular system (vasa hyaloidea propria) that filled the primary vitreous, are also present in the vitreous base.81
Teng and Chi82 found that the vitreous base posterior to the ora serrata varies in width, depending on the age of the individual. More than half of eyes from persons older than 70 years had a posterior vitreous base wider than 1.0 mm. The width increased with increasing age to nearly 3.0 mm, bringing the posterior border of the vitreous base closer to the equator. This widening of the vitreous base is believed to be most prominent in the temporal portion of the globe.83 The observation of posterior migration of the vitreous base has recently been confirmed by studies84 in England that also identified intraretinal synthesis of collagen fibrils that penetrate the internal limiting lamina of the retina and ‘splice’ with vitreous collagen fibrils. This has been proposed as the explanation for increased vitreoretinal adhesion at the vitreous base, which predisposes to rhegmatogenous retinal detachment because this is the area of strongest vitreoretinal adhesion. Although Gartner79 found no differences in the thickness of collagen fibrils in the vitreous base when comparing five eyes from humans aged 9 months and 29, 39, 61, and 71 years, he did note that there was “lateral aggregation” of the collagen fibrils in the eyes from older persons. Such aggregation at the vitreous base is similar to aging changes within the vitreous body where collagen fibril aggregation results in bundles of parallel collagen fibrils. These aging changes at the vitreous base could also contribute to increased traction on the peripheral retina and play a role in the development of retinal tears and detachments.
Surgical Anatomy of Retinal Tears and Detachments
Retinal Tears
The aforementioned age-related posterior migration of the posterior border of the vitreous base does not extend posteriorly in a continuous line, which is the configuration of the posterior border of the vitreous base in a young person, but in a curvilinear, undulating pattern. Traction on the retina along this line can create the exact “horseshoe” configuration often seen in peripheral retinal tears. It is known that in the vitreous base there are collagen fibrils oriented perpendicular to the wall of the eye81,85,86 with insertions anterior and posterior to the ora serrata.65,87 The continuity of these fibrils with those of the vitreous body is an important predisposing factor for retinal tears and detachments.
At the turn of the 20th century, Best88 emphasized that every movement of the eye results in movement of the vitreous body and that this causes traction at any point of strong vitreoretinal adhesion. Leber et al.89 were perhaps the first to find that rupture of the retina can occur at sites where there is adhesion between vitreous and retina. Unusually strong vitreoretinal adhesion is found at the posterior border of the vitreous base in cases of giant tears.31 Strong vitreoretinal adhesion is also present in anomalous or pathologic situations, such as meridional folds, peripheral pigment clumps, retinal rosettes, granular patches, and progressive latticelike degeneration.31,90,91
In lattice degeneration, discrete oval areas of retinal thinning are associated with localized vitreous liquefaction, separation of the overlying vitreous, and increased vitreoretinal adhesion at the margins of the degenerated areas.92 Retinal breaks are found at these margins and posterior to the areas of lattice degeneration. In this disorder, however, retinal tears are relatively infrequent as compared with atrophic retinal holes. Clinical studies93,94 found retinal tears in only 1% of eyes with lattice degeneration, whereas 16.3% to 18.2% of lattice lesions had atrophic retinal holes. The risk of retinal tears is greater when the area of lattice degeneration is located juxtabasal or extrabasal, relative to the vitreous base.95,96 The retinal tears are believed to result from aggregated vitreous fibrils inducing traction on the retina.97 Obliterative fibrosis of the blood vessels in areas of lattice degeneration is present in only 11.9% of lesions94 and is seen as a “lattice-wicker” of white lines, for which the condition is named. The presence of this vascular anomaly has led to the hypothesis that retinal circulatory abnormalities are the primary cause of this condition.98 According to this theory, vitreous changes are secondary and only important in the subsequent development of retinal tears as a local phenomenon. Overall vitreous liquefaction99 and PVD100 may not be important contributing factors in this local traction, but may be important in the subsequent development of retinal detachment.101
Schepens92 first described the clinical appearance of “white-without-pressure” when a geographic area of whiteness is present in the peripheral retina. When this appearance is present only during scleral indentation it is termed “white with pressure.” Schepens attributed this appearance to peripheral vitreoretinal traction and, in a subsequent report,102 it was considered that these findings predisposed to peripheral retinal tears. Daicker103 proposed that this appearance results from “collagenic” formations in the peripheral retina, whereas Gartner85 suggested that they were caused by irregularities of the internal limiting lamina of the retina. Watzke104 performed clinicopathologic correlation of a case with this finding and described that the lesion was caused by portions of the vitreous cortex that remained attached to the retina after PVD. This, therefore, would represent a variant of vitreoschisis (splitting of the vitreous cortex), in this instance at the peripheral fundus. Green105 described that the appearance of these lesions results from incident light (from the ophthalmoscope) that is tangential to more dense bundles of vitreous base collagen fibrils. All of these interpretations would seem to be consistent with the concept that these areas are at greater risk of developing retinal tears as a result of unusual vitreous structure at the vitreoretinal interface in these locations. Byer,106 however, does not believe that this ophthalmoscopic appearance has any diagnostic or prognostic significance.
Because the vitreous base is the site of strongest vitreoretinal adhesion, it is here, usually at the posterior border, that vitreoretinal traction causes peripheral retinal tears. Green105 has reviewed clinical and postmortem studies on the prevalence of peripheral retinal tears. Clinical findings ranged from a prevalence of 0.59% to 7.2%, whereas autopsy studies showed a prevalence from 3.3% to 8.8%. Other studies have focused on the relationship between PVD and peripheral retinal tears. Autopsy studies86 found that PVD is associated with retinal breaks in 14.3% of all cases. Clinical studies36,107,108,109 found retinal tears in 8% to 15% of eyes with acute PVD. In the presence of high myopia (> -6 D), PVD is associated with peripheral retinal breaks in 11.1%.110 This could be partly related to the significant increase of liquid vitreous observed in experimentally induced myopia.111 In patients with high myopia who had uncomplicated cataract extraction (presumably by intracapsular techniques), the prevalence of retinal breaks after PVD was as high as 16.2%.112 It is surprising that the prevalence of retinal tears in high myopia is not greater than reported, particularly in those cases that underwent cataract extraction. Further studies may be needed to confirm these observations. If this finding is correct, however, it may point out that changes within the vitreous that induce peripheral retinal traction only result in retinal tears in the presence of pre-existing irregularities at the vitreoretinal interface. Thus, although there may be a higher incidence of liquefaction and PVD in myopia, the vitreoretinal interface may not be similarly abnormal; consequently, peripheral retinal traction does not result in a significantly higher incidence of retinal tears in these patients. Once a retinal tear develops in a myopic eye, however, it may be more likely to produce a retinal detachment because of the biochemical and rheologic abnormalities in the liquefied myopic vitreous.
Retinal Detachments
In a study of 100 patients with bilateral surgical aphakia (presumably intracapsular) and rhegmatogenous retinal detachment in one eye, Hovland113 found that 26% eventually developed peripheral tears and retinal detachments in the fellow eye. In this study, the absence of PVD in the fellow eye at the time of retinal detachment in the first eye was the poorest prognostic sign for the fellow eye. Bradford et al.114 found that in those rhegmatogenous retinal detachments that developed within 6 months after cataract surgery, equatorial tears were significantly more common than in detachments that occurred 2 or more years after cataract extraction. Because this is not the typical profile of an “aphakic” retinal detachment, Bradford et al. hypothesized that these retinal tears occurred at the time of PVD and were caused by the presence of anomalous vitreoretinal adhesions. Furthermore, because this appearance is no different from phakic rhegmatogenous retinal detachments, cataract surgery was probably not an important factor in these cases, although it may have precipitated the PVD as a result of biochemical changes within the vitreous. These authors concluded that the small anterior tears that cause retinal detachments long after cataract extraction result from chronic vitreoretinal traction at the vitreous base, rather than acute PVD.
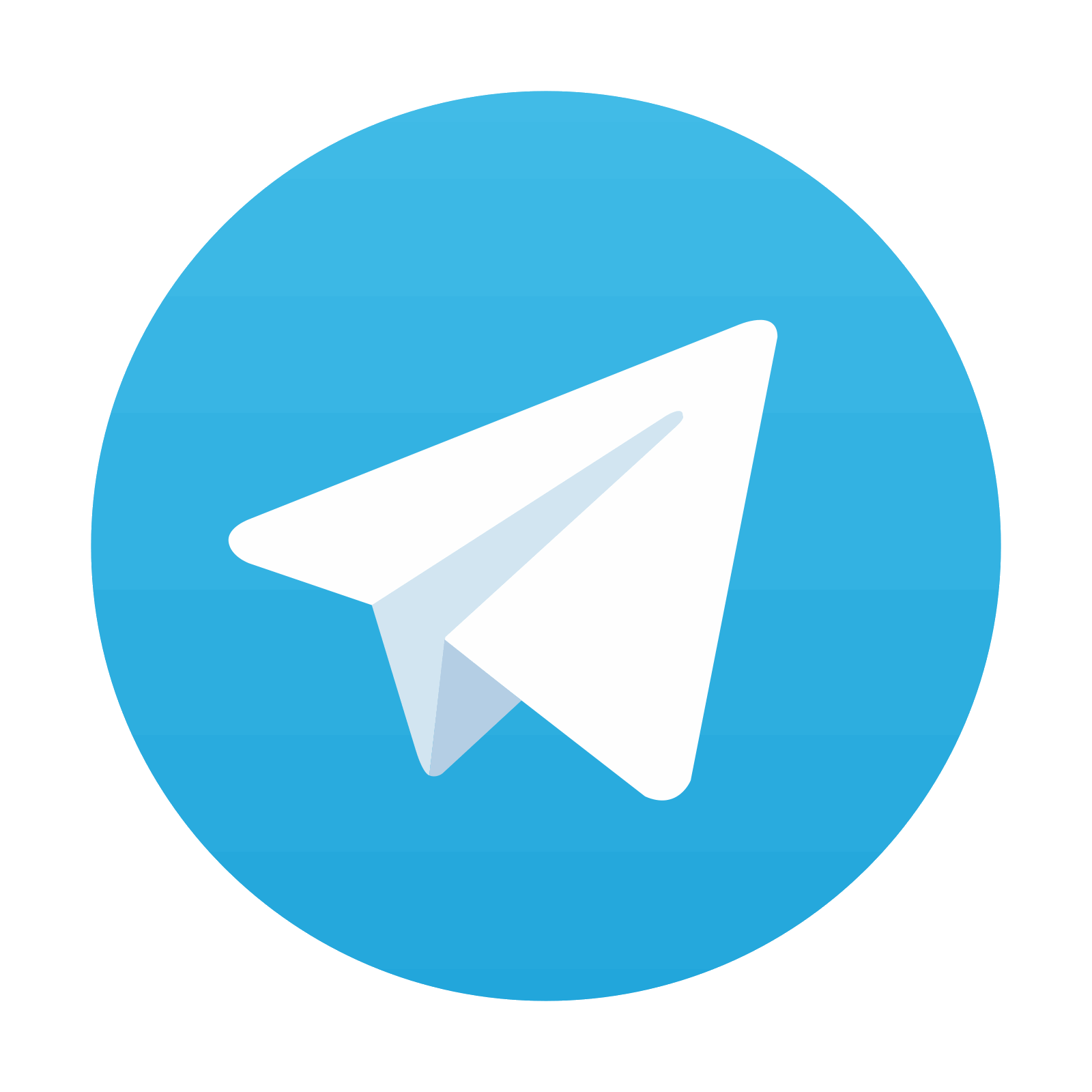
Stay updated, free articles. Join our Telegram channel
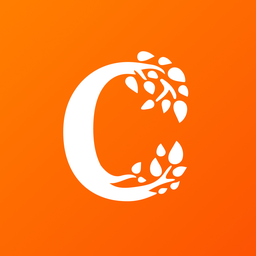
Full access? Get Clinical Tree
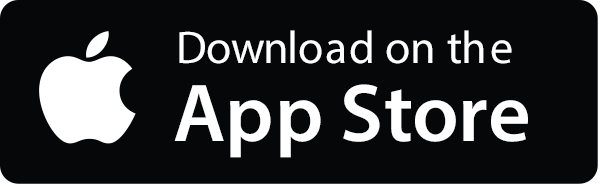
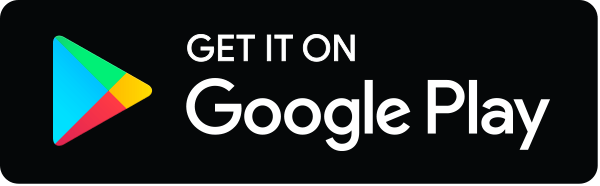
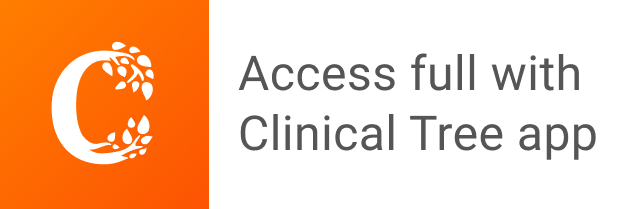