(1)
Department of Ophthalmology and Visual Sciences, University of Iowa Hospitals and Clinics, Iowa City, IA, USA
Vascular disorders of the retina and choroid are common. For understanding their pathogeneses, it is essential to know the pathophysiology of various factors, which influence retinal and choroidal circulation and blood flow.
Pathophysiology of Factors Influencing the Retinal and Choroidal Blood Flow
The following formula is used to calculate the ocular blood flow:
Perfusion pressure can be calculated in two ways:
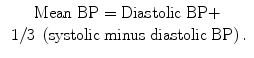

1.
Perfusion pressure = mean arterial blood pressure (BP) minus venous BP in the retinal/choroidal vessels.
2.
Perfusion pressure = mean arterial BP in the retinal/choroidal vessels minus intraocular pressure (IOP). Normally the pressure in the central retinal vein at the optic disc is slightly higher than the IOP, so that for all practical purposes, IOP is usually a good index of the ocular venous pressure.
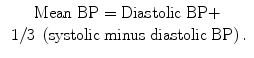
Thus, the blood flow depends upon three factors: (a) resistance to blood flow, (b) BP, and (c) IOP. I would like to discuss at some length the pathophysiology of these three factors, as well as of some other factors influencing the retinal and choroidal blood flow.
Resistance to Blood Flow
This plays a very important role in the blood flow. The resistance to blood flow, according to Poiseuille’s law, is inversely proportional to the fourth power of the radius of the vessel and directly proportional to blood viscosity and the length of the vessel. Therefore, two factors can influence resistance to blood flow in the retina and choroid. These are (a) the state and caliber of the vessels feeding the retinal and choroidal circulation and (b) rheological properties of the blood.
The State and Caliber of the Vessels Feeding the Retinal and Choroidal Circulation
The retina is supplied by the central retinal artery and sometimes also by the cilioretinal artery. The choroid is supplied by the posterior ciliary arteries (PCAs). The caliber of these arteries is controlled by the following factors.
Autoregulation of Blood Flow
It is well established that retinal circulation has autoregulation. However, choroidal circulation is mainly controlled by sympathetic innervation and is not autoregulated [1]. Kaya et al. [2] in a study of 10 healthy young male subjects reported that their data indicate that the vascular bed distal to the central retinal artery shows better autoregulatory properties as compared to the PCAs. Kiel et al. [3–5] in rabbits and Yu et al. [6] in guinea pigs have reported that choroidal blood flow is autoregulated; however, in both studies, the retina was avascular, which is not the case in the human; that most probably explains the difference in the findings of autoregulation in the choroidal vascular bed between the human and species with avascular retina. Studies in the newborn have shown that choroidal autoregulation is almost completely absent [7–9].
A study in normal subjects showed retinal vascular autoregulation in response to change of position from sitting to reclining [10]. Rose et al. [11] reported that retinal arterioles and venules provide equally reproducible results for autoregulation studies and that arteriolar pulsatility profiles provide additional useful information regarding vascular resistance. In normal subjects, after exercise, autoregulation is sufficient to compensate the increase in blood pressure and maintain a stable retinal blood flow [12].
What Is Autoregulation of Blood Flow?
Blood flow autoregulation is the inherent capability of a tissue to maintain its blood flow relatively constant despite variation in perfusion pressure [13]. This means the blood flow is actively regulated by metabolism through the action of local factors that modulate the tone of the retinal vascular resistance. Thus, autoregulation of blood flow is due to alteration in resistance to blood flow, and that in turn is due to changes in the tone of the blood vessels. It is generally thought that the terminal arterioles regulate the resistance to flow, i.e., they dilate to increase the blood flow when the perfusion pressure falls and constrict to reduce the blood flow in arterial hypertension. Since there is a limit to how far the terminal arterioles can constrict or dilate, the autoregulation operates only within a certain critical range of perfusion pressure and breaks down when the perfusion pressure goes below or above this critical range (Fig. 10.1). Therefore, contrary to the general belief, the presence of autoregulation does not automatically regulate the blood flow in a tissue at all times.
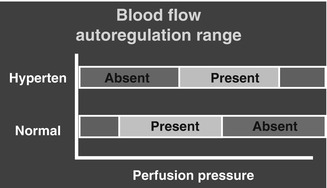
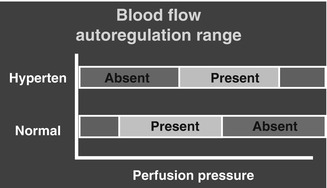
Fig. 10.1
A diagrammatic representation of blood flow autoregulation range at different perfusion pressures in normal persons and in hypertensives (Hyperten). Red (absent) and green (present) denote absence or presence of the autoregulation
Anderson and his colleagues have discussed the role of pericytes in the capillaries in regulation of the blood flow autoregulation by virtue of the presence of contractile proteins actin and myosin [14, 15]. Retinal capillary pericytes, by the endothelium-relaxing factor nitric oxide (NO), can modulate the tone of capillaries and thus have the potential to influence blood flow in them [16]. Discontinuities in the vascular basement membrane allow the pericytes to make direct contact via gap junctions with the endothelial cells on their abluminal aspect. Therefore, it has been suggested that there is a vessel wall cross talk between pericytes and the microvascular endothelium to maintain a smooth and uninterrupted retinal blood flow [17].
What Is the Exact Mechanism Responsible for Blood Flow Autoregulation?
Autoregulation works by changing perfusion pressure by altering the resistance in the terminal arterioles and capillaries. The exact mechanism of how it is achieved is still not clear. There is no autonomic nerve supply to retinal vessels and it still shows an efficient autoregulation, which indicates that it is mainly influenced by local factors discussed below. On the other hand, the choroidal vascular bed has a rich autonomic nerve supply and has no autoregulation.
Briefly, there are three hypotheses to explain mechanisms of blood flow autoregulation:
(a)
Metabolic Hypothesis: According to this, local arteriolar smooth muscle tone is regulated by local concentration of metabolic products. Blood gases modulate the arterial tone because it has been shown that increase of PaO2 (hyperoxia) produces marked vasoconstriction of the inner retinal arterioles [8, 18–27]. A study [28] showed that inhaling 100 % oxygen compared with room air resulted in constriction of retinal vessels and decrease in retinal blood flow. Conversely a decrease in PaO2 (hypoxia) produces vasodilation of the retinal arterioles [19, 29]. Accumulation of metabolites due to hypoxia or lack of ability to wash out such metabolites causes vasodilation [30–33]. Elevation of pCO2 does seem to be one of the factors in the vasodilation of arterioles [34, 35], and that markedly influences the peripheral resistance [36, 37] – the effect is most marked in the arterioles [38]. Anderson and Davis [39] found that an increase in pCO2 caused acidosis of the medium and relaxation of the pericytes in a reversible, concentration-dependent manner. These findings suggest that the regulation of blood flow to meet local metabolic needs does not reside entirely in the arterioles and precapillary sphincters but at least in part in the capillary bed through the contractile properties of pericytes. The local pCO2 is one of several potential indicators of whether or not local blood flow is adequate for local tissue needs. In contrast to the retinal vasculature, choroidal blood flow decreases during hyperoxia [8, 40].
(b)
Myogenic Hypothesis: According to this, the rise of intravascular pressure causes vasoconstriction [41–47], because stretching of the vessel wall is counteracted by vasoconstriction in the arterioles. The myogenic response is inhibited by calcium channel inhibitors, as has been well demonstrated by the use of calcium channel blocker drugs in treatment of arterial hypertension. Endothelial derived vasoactive agents (discussed below) are thought to be most probably responsible for initiating the myogenic contraction [45–47].
(c)
Neurogenic Hypothesis: Vessels in the retina have no autonomic nerve supply but still have autoregulation. The choroid, by contrast, is richly supplied by the autonomic nerves [48–65] and yet has no appreciable autoregulation in man. This suggests that neurogenic factors do not play much role in autoregulation.
Pournaras et al. [66] have recently reviewed the autoregulation of retinal blood flow. Arterial tone is modulated by release of vasoactive substances by the vascular endothelium, and these are vasodilators (nitric oxide, prostacyclin) and vasoconstrictors (endothelins, angiotensin II, thromboxane-A2, and prostaglandin H2). Vascular tone is determined by the balance between the vasoconstrictors and vasodilators (Fig. 10.2).
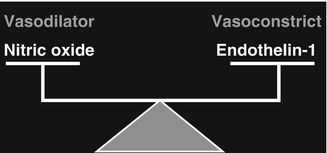
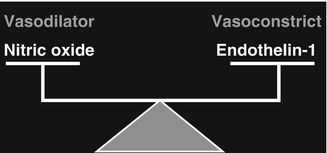
Fig. 10.2
A diagrammatic representation of balancing role of nitric oxide and endothelin-1 in modulating local vascular tone
Autoregulation of retinal blood flow is probable due to a balanced contribution of both myogenic and metabolic components [67], involving the interaction of factors released by the retinal metabolism and the vascular endothelium [68]. An increase in transmural pressure produces a myogenic response in isolated retinal arteries [43, 67]. Role of blood gases is discussed above. Extracellular lactate leads to contraction or relaxation of the vessel wall, according to the metabolic needs of the tissue [69].
What Is the Range of Perfusion Pressure Over Which Retinal Autoregulation Operates?
As discussed above, autoregulation operates only over a critical range of perfusion pressure, so that with a rise or fall of perfusion pressure beyond the critical range, the autoregulation becomes ineffective and breaks down (Fig. 10.1); under those circumstances the blood flow is directly proportional to the perfusion pressure. Thus, it is essential to remember that autoregulation does not protect the retinal blood flow at all times. Retinal blood flow is maintained constant over a range of 45–145-mmHg perfusion pressure in the adult [8, 70–75]. In contrast, retinal blood flow is autoregulated over a range of 45–85-mmHg perfusion pressure in newborn piglets [6]. Jeppesen et al. [44] reported that retinal autoregulation decreases in normal persons at ages above 40 years.
Breakdown of Blood Flow Autoregulation
1.
At What Level of Perfusion Pressure Does Autoregulation Break Down? This happens when the perfusion pressure goes above or below the normal level.
2.
What Causes Derangement of the Retinal Autoregulation? Several factors play a role in it, including the following.
(a)
Arterial Hypertension: Arterial hypertension causes derangement of blood flow autoregulation. The subject is discussed in detail in the section dealing with arterial hypertension below.
(b)
Vascular Endothelial Vasoactive Agents: Recent studies have shown that these agents play an important role in modulating the local vascular tone and most probably also in blood flow autoregulation (see below).
(c)
Other Causes: There may well be other, undiscovered causes of derangement of the autoregulation in the retinal circulation. We still have much to learn. Defective autoregulation of blood flow in the retina has been shown in old, arteriosclerotic, atherosclerotic, hypertensive rhesus monkeys [76, 77].
Endothelial Derived Vasoactive Agents
From the vasomotor and blood flow point of view, one of the most important discoveries has been the characterization of the role of various endothelial derived vasoactive agents. These agents are formed by vascular endothelium.
The Various Known Endothelial Derived Vasoactive Agents
These are prostanoids, nitric oxide (NO), endothelins, angiotensins, oxygen free radicals, smooth muscle cell hyperpolarization, thromboxane A2, and other agents [78–81]:
(a)
Prostanoids: Consist of prostacyclin (a vasodilator) and antiplatelet aggregator and profibrinolytic agents.
(b)
Nitric Oxide (NO): This is the “endothelial derived relaxing factor” and is synthesized from L-arginine by NO synthetase; the latter’s activity is modulated by Ca2+ influx into the cell. NO is soluble and has small size molecules; therefore, it diffuses across membranes over large distances in the order of several hundreds of micrometers, and that implies that NO produced in one cell may affect a huge number of adjacent cells [82]. It has a short half-life and is radical in nature. Its synthesis is largely dependent on the amount of nitric oxide synthase (NOS) enzyme. In the retina, NOS was identified in amacrine and ganglion cells [83–89], retinal pigment epithelium [90–92], Müller cells [88], photoreceptors [89, 90, 93], and nerve fibers in the inner and outer plexiform layers [93]. NOS activity was also found in the vascular endothelium of retinal vessels [94, 95] and pericytes [96, 97]. It was reported that retinal pericytes are involved in the control of NOS expression in retinal capillary endothelial cells [97]. There is increasing evidence that NO plays an important role in basal retinal blood flow. In choroidal blood flow, studies have shown that NO is a major factor [5, 98–104]. There is evidence that the control of vascular tone in the eye by NO depends upon the size of the vessel. For example, Haefliger et al. [105] showed that bradykinin induces a more pronounced effect on porcine ciliary arteries than on ophthalmic arteries from the same species. NO plays an important role in the maintenance and control of vascular tone in the ocular blood vessels, as well as in blood flow of the newborn and adult [8, 9, 65, 85, 106]. Thus, NO plays an important role in the control of tone of retinal vessels and autoregulation.
Jacot et al. [107] reported that NO may be a primary mediator in maintaining resting vascular tone to the choroid and anterior uvea in vivo and that NO blockade reduces the degree of compensation in the autoregulatory gain of the retinal vasculature within a specific range of ocular perfusion pressures.
(c)
Endothelins: These are the most potent vasoconstrictors and comprise endothelin-1, endothelin-2, and endothelin-3, which act via the endothelin receptors A and B. Endothelin receptor A is found on the vascular smooth muscle and B on the vascular endothelial cells. One of the ways the endothelin receptor A mediates vasoconstriction is by increased influx of calcium ions. Ceccarelli et al. [108] reported that endothelin acts on the pericytes and vascular smooth muscle cells of the retinal microvascular bed.
(d)
Renin-Angiotensin System: This has an important role in the control of arterial BP. It has been shown that this system also exists in the vessel wall and plays a significant part in vasomotor control [109–113]. Angiotensin-1 can be synthesized by endothelial cells and is converted to angiotensin-2 (a powerful vasoconstrictor) by angiotensin-converting enzyme (ACE) in the endothelial cells. ACE also inactivates bradykinin which stimulates the release of NO and prostanoids. Thus, an ACE inhibitor would decrease angiotensin-2 as well as increase bradykinin activity, the latter resulting in increased formation of endothelial derived relaxing factors – both mechanisms causing vasodilation. ACE inhibitors are used in treatment of arterial hypertension.
(e)
(f)
Mechanosensors: Endothelial cells not only regulate vasomotor function by the various endothelial derived vasoactive agents but also they can function as mechanosensors and can transduce the mechanical signals produced by the physical force of blood flow into a biochemical signal to which the vessel can respond [47], and this may be responsible for flow-dependent changes in circulation.
Causes of Abnormalities of Endothelial Derived Vasoactive Agents
Pathophysiologic alterations in endothelial cell structure and/or function occur in most major cardiovascular diseases [81]. For example, endothelial changes are seen in atherosclerosis (see below), arterial hypertension [122–124], diabetes mellitus [125, 126], ischemia [127–129], and cardiovascular diseases [81]. Available data suggest that essential hypertension decreases production of whole-body nitric oxide below the basal conditions [124]. Ischemia impairs production of NO by the endothelial cells [127–131] and may also bring on vasospasm by increased formation and binding of endothelin [132–134]. It has been shown that when the arterial oxygen falls to a critical level, the endothelial cells stop production of NO, resulting in vasoconstriction [135, 136].
Role of Endothelial Derived Vasoactive Agents in Regulation of Blood Flow
Vascular endothelium plays an important and dynamic role in vasomotor function of both macro- and microvasculatures, including maintenance of vascular tone and regulation of blood flow, and it also regulates platelet function, coagulation, and vascular growth [81]. Vascular tone depends upon a balance between the endothelial vasodilators (e.g., NO) and vasoconstrictors (e.g., endothelin) so that reduced formation of vasodilators would result in vasoconstriction and vice versa (Fig. 10.2). Therefore, endothelial cells play an important role in modulating the microvascular tone and blood flow autoregulation. In addition to vasomotor function, endothelium also plays a role in regulation of fibrinolysis because plasminogen activators and inhibitors are synthesized by the endothelium [137, 138] and in thrombus formation by affecting the platelet aggregation, adhesion, and other properties through prostacyclin, NO, and other agents [81].
Atherosclerosis and Endothelial Derived Vasoactive Agents
Atherosclerosis is a common cause of ischemic disorders in the body, including the eye. In atherosclerosis, endothelial dysfunction probably plays a critical role in vasomotor dysfunction [139, 140]. Endothelial dysfunction appears to have several causes and effects, including the following: (a) There is defective dilatation of the atherosclerotic arteries [141–144], even though the endothelium is intact, which may contribute to increases in vasoconstrictor response. (b) Vasodilatation in response to vasoactive products released by activated platelets is attenuated or reversed to vasoconstriction [145]; impaired endothelium-dependent relaxation of atherosclerotic arteries may contribute to augmented vasoconstriction by serotonin released by platelet aggregation on atherosclerotic plaque [146]. (c) Increased destruction of NO may play an important role in impairment of endothelium-dependent relaxation in atherosclerotic arteries [147]; thus, in atherosclerosis NO formation may be normal or even increased [148] but increased degradation of NO may result in impaired vasodilatation. (d) Although atherosclerotic lesions are confined to arteries, abnormal endothelial function is also seen in microcirculation in atherosclerotics [149]. (e) NO reduces adherence of platelets and leukocytes to endothelium [150], but, in atherosclerotic arteries, reduced formation or increased destruction of NO by the dysfunctional endothelium may increase adherence of platelets and consequently increase release of serotonin.
There is also evidence that changes in the smooth muscles of atherosclerotic arteries also play a role in vasomotor dysfunction. (a) They make them less responsive to NO, which increases vasoconstriction [147]. (b) They cause a modest nonspecific increase in responses to several vasoconstrictor agents, including thromboxane and endothelin-1. (c) They produce a marked increase in the vasoconstrictor effect of serotonin [140, 151, 152].
Role of Endothelial Derived Vasoactive Agents in Ocular Ischemic Disorders
As far as the ocular vascular bed is concerned, Bill [49] recognized that nitrovasodilators act as vasodilators in the eye. NO is released in the retina. It is released from the endothelial cells of the blood vessels [96, 153] and in the retina by bipolar cells [154], amacrine cells [155], and ganglion cells [83]. A number of studies have reported the important role played by endothelin-1 and NO in modulating the local vascular tone and regulating blood flow in the ophthalmic, posterior ciliary, and retinal arteries and retinal vessels [65, 105, 106, 156–163]; angiotensin-2 has been reported to cause contraction of isolated human PCAs [164]. In larger ophthalmic vessels, this is due to their effect on vascular smooth muscle cells and in small vessels by their effect on pericytes. Meyer et al. [160] reported that in isolated ciliary arteries of pigs, local anesthetics impair endothelial formation of NO from L-arginine after stimulation with bradykinin; the authors postulated that local anesthetic drugs interact with vascular endothelium and cause endothelial dysfunction. Studies suggest that NO in the choroid may maintain basal blood flow [65, 106]. Arteriosclerosis, hypercholesterolemia, aging, arterial hypertension, diabetes mellitus, ischemia, and other so far unknown causes in the ocular region may be associated with abnormalities in production of endothelial derived vasoactive agents, thereby influencing the vascular resistance and blood flow [159].
It has been postulated that an imbalance of NO and endothelin-1 may be important for the development of ocular vascular complications seen in diabetes mellitus, arterial hypertension, hyperlipidemia, arteriosclerosis, and ischemia. In our studies in atherosclerotic monkeys, serotonin (5-hydroxytryptamine) caused vasoconstriction resulting in transient occlusion or impaired blood flow in the central retinal artery and/or PCA [146, 165]. We speculated that it is likely that platelets adhere to and aggregate on atherosclerotic plaques and release their vasoactive materials, including serotonin, and that the released serotonin triggers vasoconstriction by its action on the smooth muscles of atherosclerotic arteries; this serotonin-induced vasoconstriction may play an important role in the development of ischemic disorders of the retina in atherosclerosis. In our atherosclerotic study, stopping the atherogenic diet abolished or markedly improved the serotonin-induced vasoconstriction within a few months.
Miscellaneous Experimental Vasomotor Studies
Flicker has been shown to increase the release of NO in the retina [166]. In monkeys, flicker increased glucose consumption in the inner retina, most likely by increasing neuronal activity [59, 167, 168]. Kondo et al. [168] found that in anesthetized cats flickering light increased blood flow in the retina by 39 % and concluded that NO release may mediate much of the vasodilation effect of flicker and play a role in maintaining normal vascular tone.
Anderson and his group have studied, by in vitro testing, the possible role of pericytes in vasomotor function. Ferrari-Dileo et al. [169] tested in cultured bovine retinal vascular pericytes the potential for vasoactive neuropeptide receptors to affect capillary resistance. They found that circulating or locally produced vasoactive neuropeptides might affect pericyte contractile tone, with the net effect on local blood flow resulting from the effects on arteries and veins as well as capillaries. Zschauer et al. [170] reported that isoproterenol produced relaxation of pericytes in a dose-dependent manner and low concentrations of the nonselective beta-blockers propranolol and timolol blocked the relaxation produced by isoproterenol. By contrast, atenolol and betaxolol, as relatively selective beta-1 adrenergic blockers, had no effect on the isoproterenol-induced relaxation.
Rheological Properties of the Blood
These would be influenced by a large variety of hematologic disorders, particularly those causing increased blood viscosity.
Arterial Blood Pressure
As mentioned earlier on, one of the factors which determines the blood flow is the perfusion pressure in the retinal vascular bed, and perfusion pressure in turn depends primarily upon the arterial BP. Clinically, the BP is measured in the brachial artery. I [171] measured, by direct cannulation of the vessels, the BP in the ophthalmic artery and in the aorta in normal, healthy, adult rhesus monkeys simultaneously, and regression equations were computed to calculate the ophthalmic artery pressure from the aortic BP. These were as follows:




If there are vascular changes in the arteries feeding the retinal and choroidal circulations, i.e., in the internal carotid artery, ophthalmic artery, central retinal artery, and PCAs (produced by vasospasm, arteriosclerosis, atherosclerosis, vasculitis, drug-induced vasoconstriction or dilatation, or other systemic and cardiovascular diseases), then the BP in the retinal capillaries and choriocapillaris would be much lower than that in a normal healthy condition. Thus, it is important to realize that clinical BP measurement in the brachial artery very much overestimates the actual BP in the retinal and choroidal vessels, particularly in the capillaries which play a primary role in nutrition of the retinal tissue.
Both arterial hypertension and hypotension can influence the retinal and choroidal blood flow in a number of ways.
Arterial Hypertension
Both chronic hypertension and malignant hypertension can interfere with the retinal blood flow. Blood flow in the retina may be altered by a number of mechanisms in these patients, including the following:
1.
Increased Vascular Resistance in Terminal Arterioles: This is the basic pathology in arterial hypertension and it reduces the blood flow in the capillary bed.
2.
Secondary Changes in Blood Flow Autoregulation: Arterial hypertension can interfere with the normal autoregulation of the blood flow. This may be due to the following mechanisms:
(a)
Due to Abnormalities in Endothelial Derived Vasoactive Agents: Arterial hypertension produces endothelial abnormalities (as discussed above), and those can cause abnormalities in the production of endothelial derived vasoactive agents, particularly reduced production of NO. Vascular tone depends upon the balance between the vasoconstrictor (endothelin) and vasodilator (NO) (Fig. 10.2). Decreased production of NO upsets the balance, so that the vasoconstrictor endothelin becomes overactive and results in vasoconstriction. That in turn would result in increased vascular resistance and deranged autoregulation of blood flow.
(b)
Due to Hypertension-Induced Changes in Blood Flow Autoregulation: In accelerated or malignant arterial hypertension, when the BP suddenly goes above the autoregulation range, it produces vascular damage, e.g., hypertensive fundus changes [172, 173] and hypertensive encephalopathy (Fig. 10.1). However, if the BP rises less rapidly, there is ample evidence that the various circulatory systems in the body with autoregulatory mechanisms (cerebral circulation [174–183] and coronary circulation [184]) show an adaptive phenomenon, wherein the range of autoregulation shifts to a higher level than in normal persons (Fig. 10.1). This adaptation improves a person’s tolerance of high BP but at the same time makes the individual less tolerant of low BP. There are a number of reports in which patients with malignant hypertension, who had their BP lowered precipitously to prevent severe neurological and cardiovascular complications, suffered immediate and permanent blindness or severe visual loss from anterior ischemic optic neuropathy [185–189]. There is fairly good proof that in malignant arterial hypertension, the autoregulatory mechanism is deranged in the retinal [190] and optic nerve head [191] circulation. The same holds good for the coronary circulation; a marked fall of BP in hypertensives during sleep can precipitate myocardial ischemia, particularly in patients with obstructive coronary artery disease [184]. Alderman et al. [192] found that a large reduction in BP (≥18 mmHg) induced by treatment of hypertension showed a relationship with development of myocardial ischemia. This is further suggested by studies which have shown that when arterial hypertensives are treated with antihypertensive agents, lowering BP below a critical level increases cardiac mortality and morbidity. For example, the rate of cardiac events at 75 mmHg diastolic BP is twice than that at 85 mmHg [193]. Similarly, in our 24-h ambulatory BP monitoring studies, we found that hypertensives on oral arterial hypotensive therapy (with beta-blockers, calcium channel blockers, and/or ACE inhibitors) showed a significant association between progressive visual deterioration and nocturnal hypotension, particularly in patients with anterior ischemic optic neuropathy [194, 195]. Thus, nocturnal arterial hypotension in hypertensives could contribute to cardiac, cerebral, retinal [194, 196], and optic nerve head ischemia [194, 195, 197], because of defective autoregulation produced by hypertension in these organs.
3.
Due to Direct Effect of Vasoconstrictor Agents on the Choroidal Blood Vessels: Malignant arterial hypertension can interfere with choroidal blood flow by the following mechanism. Angiotensin and other circulating vasoconstrictor agents in malignant arterial hypertension leak out of the choriocapillaris into the choroidal extravascular tissue fluid. These vasoconstrictor agents produce vasoconstriction in those vessels by their direct action on the choroidal vessels, and that can interfere with the choroidal blood flow and produce choroidal and retinal pigment epithelial ischemia [198].
Arterial Hypotension
In the retina with defective autoregulation, a fall of BP below a critical level must decrease its blood flow (Fig. 10.1). A fall of BP may be due to systemic or local hypotension:
(a)
Systemic Arterial Hypotension: A frequent occurrence of this hypotension is during sleep (i.e., nocturnal arterial hypotension – Figs. 10.3 and 10.4) [173, 194, 195, 201] caused by intensive antihypertensive medication in arterial hypertension or other cardiovascular diseases (with beta-blockers, calcium channel blockers, and/or ACE inhibitors), particularly when administered in the evening or at bed time (Fig. 10.5) [173, 194, 195]. Less common causes include massive blood loss or shock. Studies have shown an association between nocturnal arterial hypotension and development of central retinal artery occlusion [196], especially in those with ocular ischemic syndrome [202].
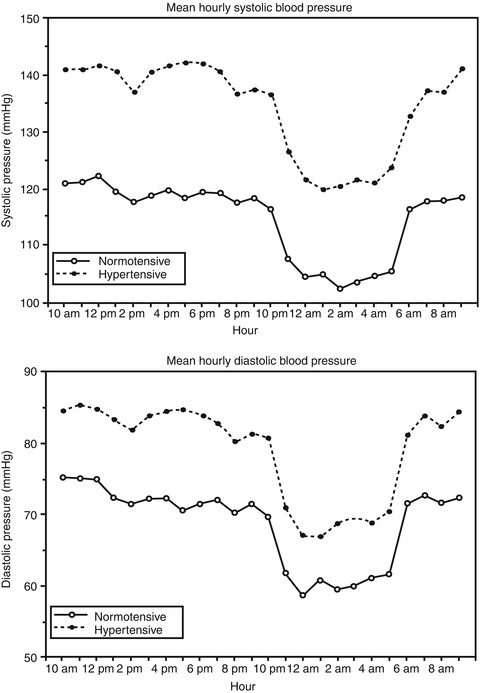
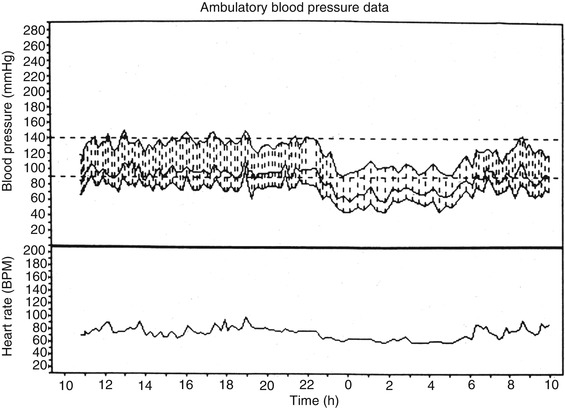
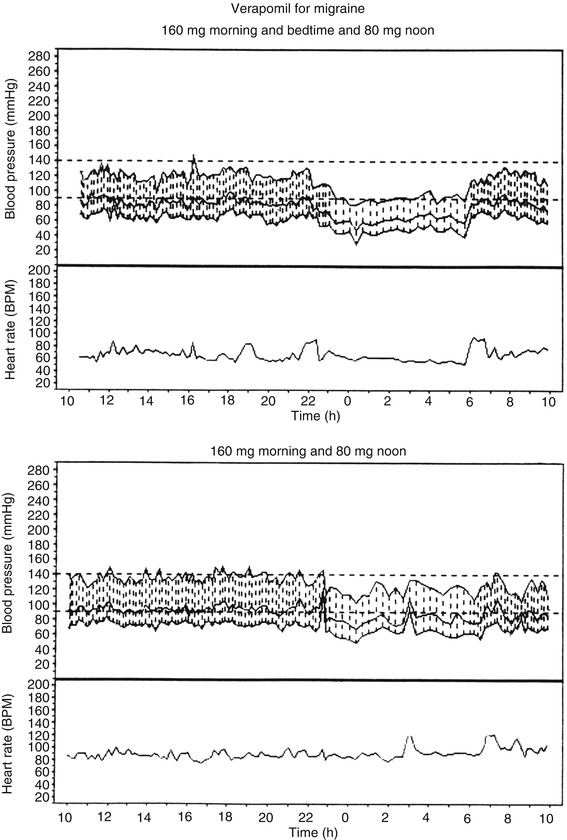
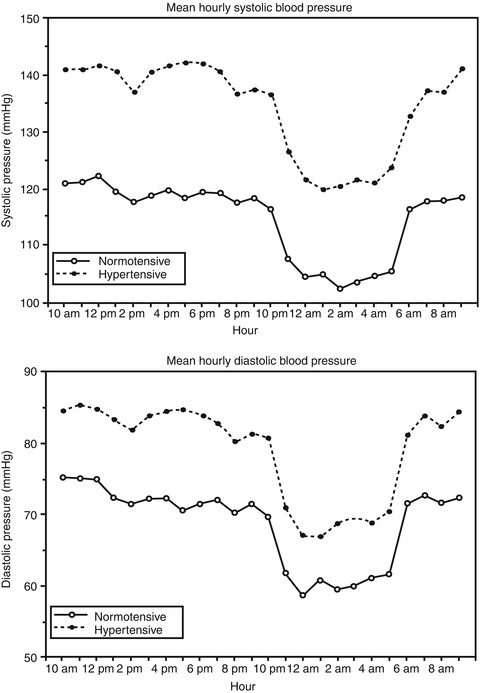
Fig. 10.3
Mean hourly systolic and diastolic blood pressures over a 24-h period in patients with normal blood pressure (normotensive) and hypertension (hypertensive) (Reproduced from Hayreh et al. [194])
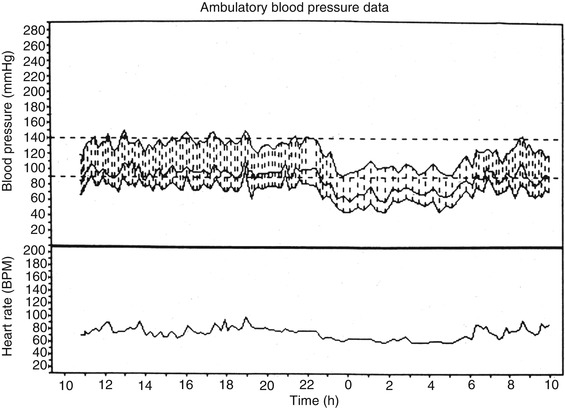
Fig. 10.4
Ambulatory blood pressure and heart rate monitoring records (based on individual readings) over a 24-h period, starting from about 11 AM, in a 58-year-old woman with bilateral non-arteritic anterior ischemic optic neuropathy and on no medication. The blood pressure is perfectly normal during the waking hours, but there are periods of marked nocturnal arterial hypotension during sleep (Reproduced from Hayreh et al. [195])
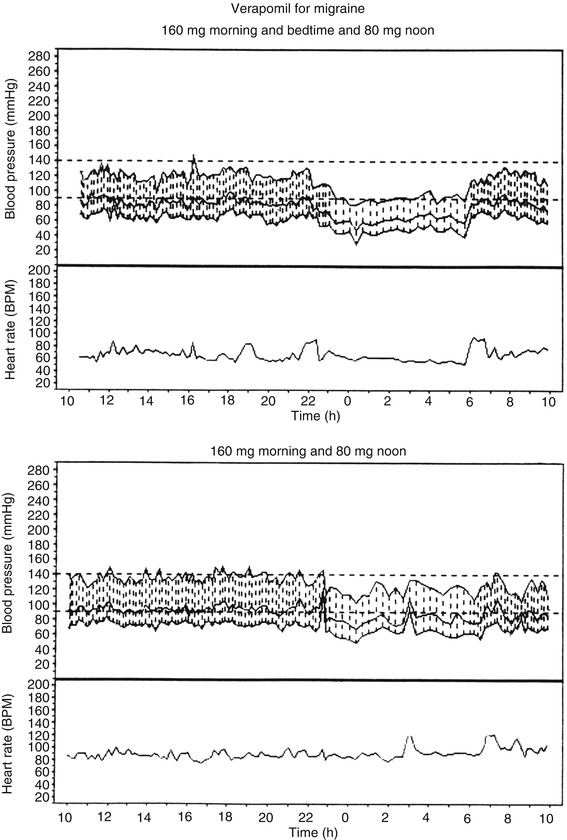
Fig. 10.5
Ambulatory blood pressure and heart rate monitoring records (based on individual readings) over a 24-h period, starting at 10 AM, of a 63-year-old woman who developed normal tension glaucoma while on regimen of verapamil hydrochloride for migraine. The upper record shows that when she was taking verapamil at bedtime, there was a marked degree of nocturnal hypotension, which improved markedly on stopping the bedtime dose, as seen in the lower record (Reproduced from Hayreh et al. [195])
(b)
Local Arterial Hypotension: Instead of or in addition to the systemic arterial hypotension, there may be a regional fall in the mean BP in the ocular blood vessels. This may be due to narrowing of the regional arteries, such as the internal carotid [202], ophthalmic, and central retinal artery or one or more of the PCAs. Under such circumstances, brachial BP measurement would grossly overestimate the BP in the ocular vessels.
Intraocular Pressure
Perfusion pressure plays an important role in determining the blood flow; the perfusion pressure is equal to mean BP minus IOP. Thus, there is an inverse relationship between IOP and perfusion pressure – the higher the IOP, the lower the perfusion pressure and consequently the lower the blood flow, if the autoregulation is defective. Normally the magnitude of fluctuations in mean BP is many times greater than those in the IOP; hence, for any significant change to occur in the perfusion pressure and blood flow, mean BP is comparatively a much more important factor than the IOP. A much greater rise in IOP would be required to interfere significantly with the blood flow in healthy persons (with normal BP and autoregulation) than in persons with arterial hypotension, defective autoregulation, or other vascular risk factors.
A number of factors produce a short-term rise in IOP. Those include the following:
1.
Effect of Body Position: IOP is elevated by a supine position [203–208], usually 3–4 mmHg [203, 204, 207]. Anderson and Grant [205] found considerable interindividual variation in the rise of IOP in a recumbent position compared to sitting; there was no change in 30 %, 1 mmHg rise in 22.6 %, 2 mmHg rise or fall in 87 %, and more than 2 mmHg in 13 %. Maximum rise was 11 mmHg and maximum fall 8 mmHg; IOP changes were greater in glaucoma patients on therapy than in those on no therapy. Tsukahara and Sasaki [206] found a mean difference in IOP between sitting and supine positions in normal persons of 5.6 ± 1.71(SD) mmHg.
2.
Circadian Variation in IOP: This is a well-established phenomenon; the literature on the subject has recently been reviewed by Zeimer [209]. Available evidence in the literature indicates that although there is a marked variability in the time of the day when the IOP peaks, in normal persons it most often peaks early in the morning and decreases as the day progresses. I have plotted diurnal curves in more than two thousand persons over the years, recording IOP every 3 h between 7 AM and 10 PM with a Goldmann tonometer, and have found that it is generally highest early in the morning and lowest late in the evening, although infrequently other variations are seen. I have seen patients with IOP almost 100 % higher early in the morning than later on in the afternoon or evening. Such a marked circadian change of IOP may be completely missed on routine clinic visits.
3.
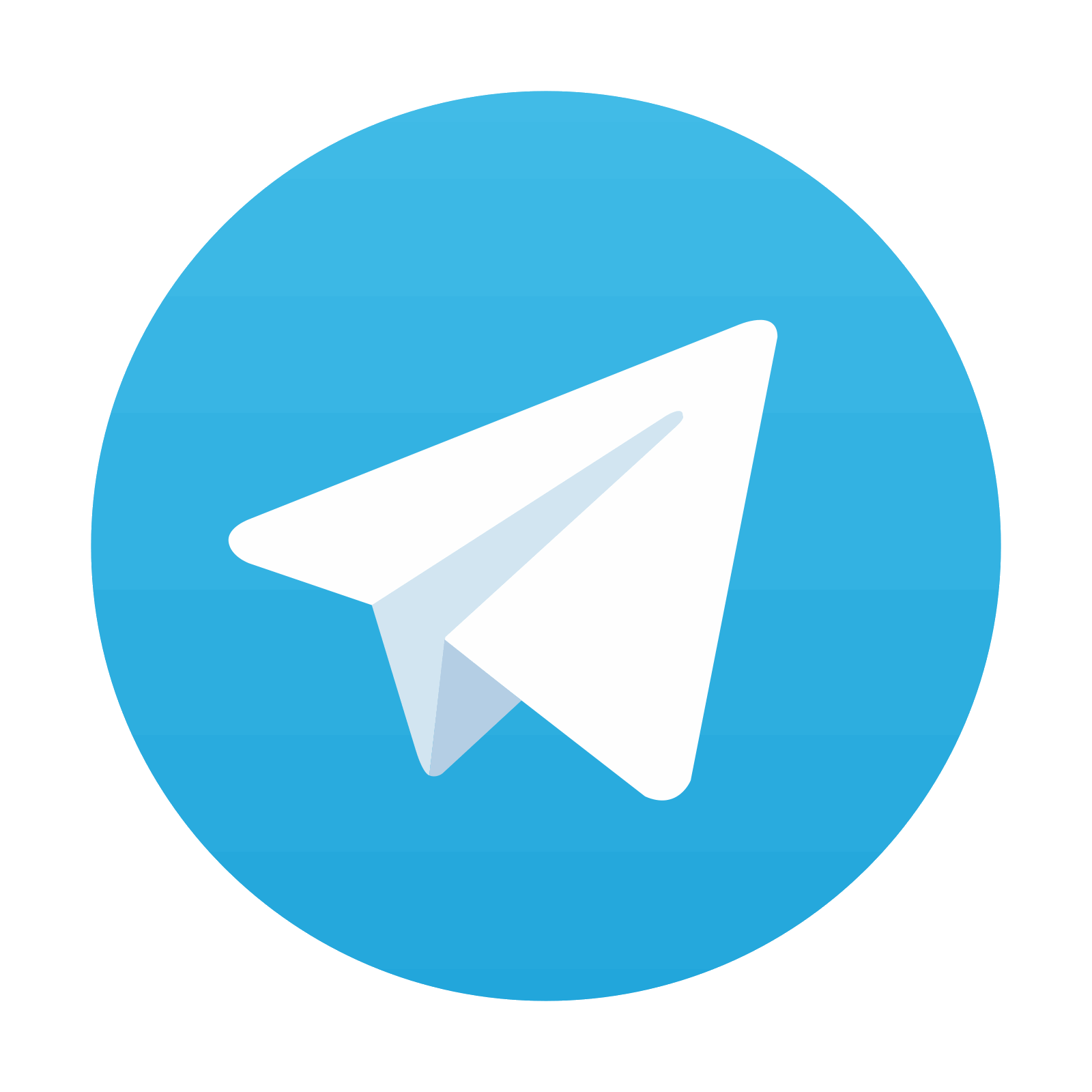
Effect of Sleep: A number of studies have investigated the effect of sleep on the IOP and have found IOP higher during sleep than during waking hours [210–213]. Brown et al. [211] found that IOP was significantly higher during sleep than during wakefulness; it was 3.45 mmHg higher after 30 min of sleep, increasing thereafter to 6.41 mmHg above baseline after sleep of up to 4 h. Buguet and Romanet [213] also found that IOP was significantly lower during the day than at night – the rise was sharp at onset of sleep in young persons, but in the elderly population, increase in IOP was more gradual throughout the night. Day and night variation in IOP was significantly related to the state of sleep/wakefulness. IOP was lower during wakefulness than during light sleep, slow-wave sleep, and rapid eye movement (REM) sleep. There was a significant difference between slow-wave sleep IOP and REM sleep IOP. IOP was highest when subjects were awakened from slow-wave sleep, which is predominant at the beginning of the night. IOP during REM sleep was lower than slow-wave sleep and not related to the eye movements but most probably to miosis occurring at that time. Lowest IOP occurred upon awakening from REM sleep, and highest upon awakening from slow-wave sleep. Wildsoet et al. [212] found that the amount of rise in IOP during sleep was reduced by sleeping in bright light as compared to sleeping in the dark. Brubaker [214] found that the rate of aqueous flow through the anterior chamber is highest during morning hours and slightly lower during afternoon hours, and the rate during sleep is approximately one half of that during the morning. The exact mechanism of the circadian variation in the IOP still remains unclear, but it has been found to be independent of the rise due to a supine position; it has been speculated that it is due to plasma cortisol level, melatonin level, or other autonomic or humoral mechanisms.
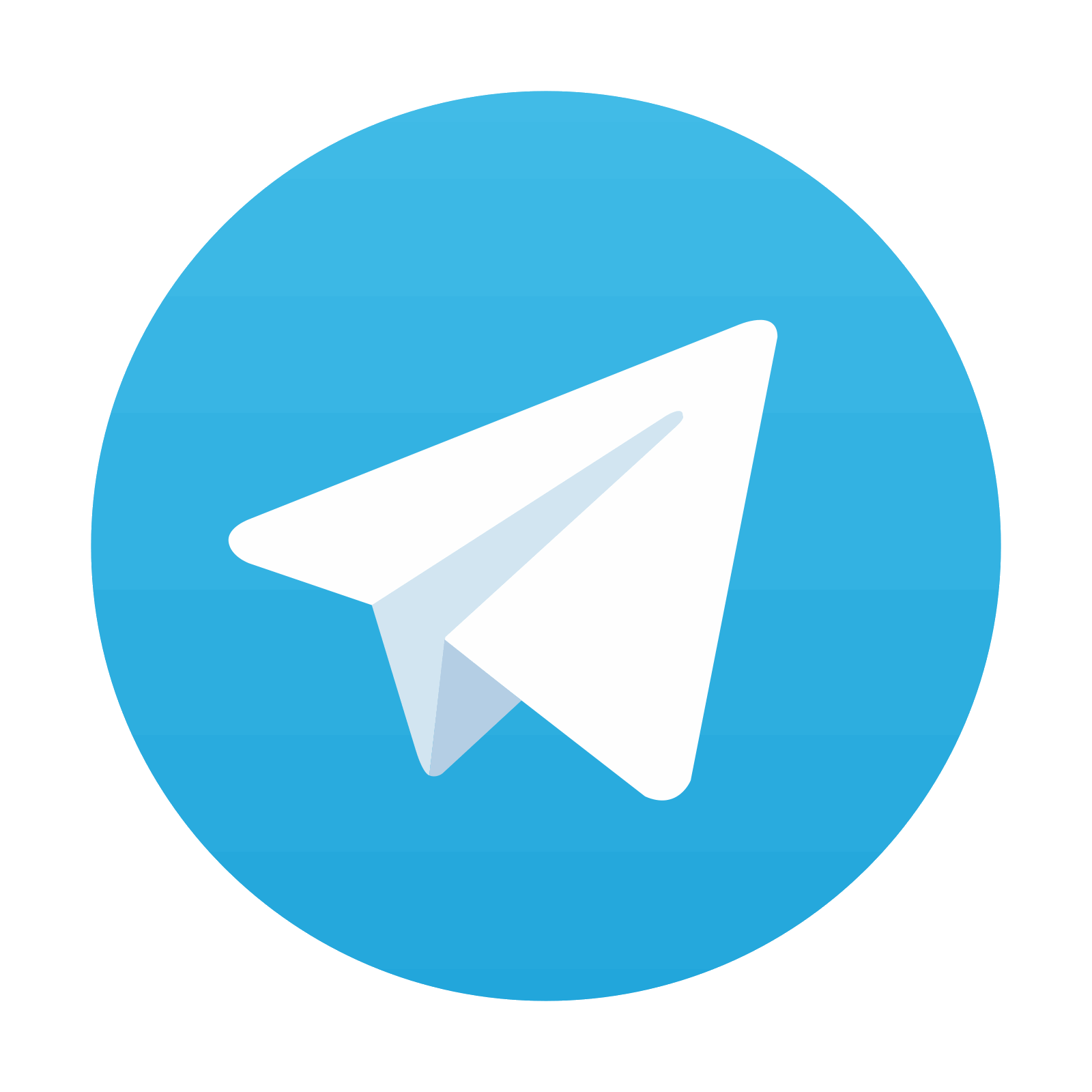
Stay updated, free articles. Join our Telegram channel
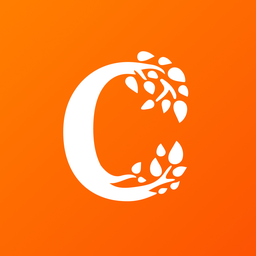
Full access? Get Clinical Tree
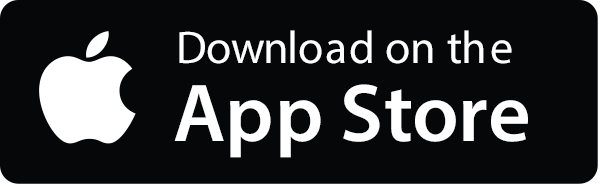
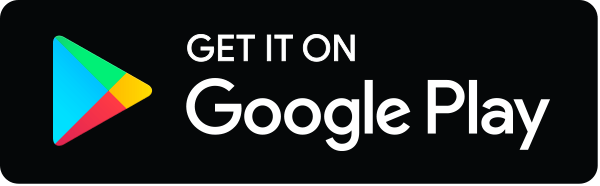
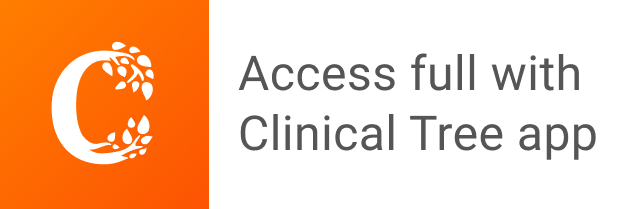