Physiology of the Lens
Nicholas A. Delamere
The lens is a vital component of the transparent pathway between the outside world and the retina. Together, the lens and cornea comprise the refractive mechanism of the eye. It is the lens, however, that adds the important element of variable focus. Because the shape of the lens can be changed, thus altering its focal length, it is possible for the eye to switch focus from a distant to a near object. To function properly, the lens must understandably be transparent and this requires considerable tissue specialization. Perhaps the most immediately apparent specialization is the lack of capillaries, veins, and nerves. The lens exists in isolation from other tissues, suspended by ligaments (zonules of Zinn) in the fluid-filled interior cavity of the eyeball (Fig. 10.1). Other, less apparent, specializations include the tight, highly ordered packing of lens cells, the loss of organelles in most lens cells, and the abundant expression of cytoplasmic proteins called crystallins. One consequence of these specializations is the remarkably long life span of lens cells and their constituents. Once made, lens cells are retained for the life of the individual. Because fully differentiated lens cells are unable to synthesize proteins and lipids, defective or damaged biomolecules cannot be replaced. Instead, the lens appears to be extraordinarily efficient at preventing protein and lipid damage. Oxidative deterioration is kept at a manageable level for decades. This feat is all the more remarkable when considering that for two-thirds of each day, potentially damaging light rays pass through the lens.
Focus and Accommodation
At rest, the lens in the normal eye focuses images of distant objects on the retina. It acts in much the same way as a biconvex optical glass lens (Fig. 10.2). Thus, the refractive power of the lens is a function of the curvature of the lens surfaces as well as the refractive index of the cell mass.1 In humans, the refractive index is 1.408. The closely packed, highly ordered, array of lens cells is essential for optical quality and it has been shown that even small defects in lens fiber cell anatomy can cause detectable spherical aberration (variability of focal length).2 Although the lens is transparent throughout, the pioneering work of Gullstrand at the beginning of the 20th century revealed that the refractive index is not uniform.1 Being more dense, the lens nucleus has a higher refractive index than the cortex. As measured by optical coherence tomography, the refractive index of the nucleus could perhaps be even higher than previously thought.3 The different refractive index in the nucleus and cortex gives the lens a total refractive power of the lens that is greater than if the refractive index was uniform throughout. Although from an anatomic standpoint the cortex blends into the nucleus, distinct zones of optical discontinuity may be seen by slit-lamp biomicroscopy. The zones are believed to correspond with various periods during the development and continual growth of the lens.
For the eye to focus the image of a near object on the retina, there must be an increase in ocular refractive power. The mechanism that achieves this change of refractive power is accommodation, and the eye at rest is said to be unaccommodated. In humans, the ability of the eye to alter focus is facilitated by a change in the shape of the lens (Fig. 10.3). There is no change in refractive index of the lens, curvature of the cornea, or length of the eyeball.4 The mechanism of accommodation varies among species5,6 and not all animals accommodate. Some fish retract their lenses to focus on distant objects, whereas in snakes and frogs and many mammals, the position of the lens moves forward for near vision. In certain other fish and horses, the retina is tilted so that the lens-retina distance alters generally in a dorsal-ventral plane. In the human, the accommodative mechanism involves the ciliary muscle, the zonules, and the lens itself. However, the role of the lens is passive in the sense that its shape change takes place as the result of contraction or relaxation of the ciliary muscle.
When the ciliary muscle is relaxed, the zonules that hold the lens in place are under tension, exerting a centrifugal force on the lens equator that causes the lens to take on a flattened form. Contraction of the ciliary muscle makes it shorten and move both forward and toward the equator of the lens. As a result, the zonules become less taut, their pull on the lens capsule is reduced, and the lens changes to its relaxed, more spherical, and thus accommodated, shape. This results in an increase in the dioptric power of the lens, allowing a near object to come into focus on the retina. To shift the focus back from a near to a distant object, the ciliary muscle relaxes and the elasticity of Bruch’s membrane causes it to move posteriorly, widening the ciliary ring so that the zonules become tense once again and flatten the lens. The principle of this currently accepted theory for the mechanism of accommodation was first suggested by Helmholtz7 in 1855, and the important role of the lens capsule was emphasized by Fincham in 1937.8 More recently, the subject has been reviewed by Charman 4.
During accommodation, the principal change in the lens shape is seen at the anterior surface. The anterior surface of the unaccommodated lens has a spherical radius of curvature of about 12 mm. When the eye accommodates, the anterior surface tends to bulge centrally, attaining a radius of curvature of about 3 mm. The more peripheral anterior surface shows relatively little or no increase in curvature. Minimal changes occur in the curvature of the posterior lens surface; Fincham8 detected changes from 5.18 to 5.05 mm in one case and from 5.74 to 4.87 mm in another case for an accommodative change amounting to 8 diopters (D) The axial thickness of the lens increases during accommodation, and the diameter decreases. The increase in lens axial thickness is paralleled by a reduction in depth of the anterior chamber of the eye.
The degree that an eye can alter its refractive power between focus on a far object and a near object is called the amplitude of accommodation. The amplitude of accommodation decreases progressively with age, a condition known as presbyopia (Fig. 10.4).9 The decline in accommodative amplitude means that the near point becomes more distant from the eye and thus small objects must be held farther away to be seen clearly. It is doubtful that there is a single cause of presbyopia. Theories proposed to explain the development of presbyopia include changes in the elastic properties of the capsule, a change in the deformability of the lens cell mass, loss of elasticity in Bruch’s membrane, and weakening of the ciliary muscle.10,11,12,13,14 Little evidence supports the concept of a weakened ciliary muscle, although age-related morphologic changes are known to occur in this tissue.15 In presbyopic eyes, the ciliary muscle is still able to contract vigorously under the influence of pilocarpine.16 Presbyopia does not appear to be simply the result of changes in the rate of lens thickening or anterior chamber shallowing with age.17 Changes in the aging capsule have been studied by Fisher,18 who concluded that reduction in capsular elasticity in the senile lens is a contributing factor in presbyopia. The principal cause of presbyopia was once considered to be “lenticular sclerosis,” a progressive hardening of the lens with age so that it becomes less deformable. However, in his elegant study of lens mechanics, Fisher 19 determined age-related changes that are not entirely consistent with the timetable for the onset of presbyopia. Recently, it has been suggested that, with aging, the continuous growth of the lens alters the geometry of the relationship between the iris root, ciliary muscle, and lens in such a way perhaps to diminish zonular tension and so impair lens shape change.20
Lens Development
The ability of the lens to function so efficiently as an optical instrument depends to a large extent on its unique anatomy. To understand lens structure it is helpful to consider its embryonic development. The lens plate (or placode) arises from a thickening of the surface ectoderm of the head-fold induced by contact with the optic vesicle, an outgrowth of the forebrain; this event takes place at the 4-mm stage (4 weeks’ gestation) in human embryos (Fig. 10.5). At about 5 weeks’ gestation (5-mm stage) the lens plate begins to invaginate to form the lens pit; at the same time the optic vesicle invaginates to form the optic cup where the embryonic lens is located. The lens pit continues to invaginate, forming a structure called the lens vesicle that separates from the surface ectoderm at approximately the 9-mm stage (5 to 6 weeks’ gestation). A thin lens capsule, the basal lamina, develops around the cell mass at about week 6. Also at around 6 weeks’ gestation, the embryonic lens is enveloped by a delicate vascular system, the tunica vasculosa lentis, which is most extensive at 10 to 12 weeks’ gestation and is supplied by the hyaloid artery of the retina. These blood vessels presumably cater to the nutritional needs of the developing lens.
The cells of the lens vesicle are positioned so that their apical surfaces face inward and their basal surfaces are in contact with the lens capsule. The cells at the posterior of the lens vesicle elongate and by approximately the 16-mm stage (7 weeks’ gestation) they have grown to form primary lens fibers that fill the cavity of the lens vesicle. The primary lens fibers eventually lose their nuclei and organelles but what remains of each cell is retained throughout life as the embryonic nucleus of the lens.
Whereas the cells at the posterior portion of the embryonic lens grow to become primary lens fibers, the cells at the equator have a different fate. They divide and give rise to new cells. In most cases, the newly formed cells differentiate and elongate to form secondary lens fibers. A few of the newly formed cells probably remain as undifferentiated epithelial cells, populating the anterior epithelial monolayer as the lens grows. The equatorial or germinative zone of the anterior lens epithelium continues to divide and produce secondary lens fibers throughout life. It is the only region of the adult lens where the cells remain capable of mitosis. Neither lens fibers nor the central anterior lens epithelium undergoes cell division.
As the cells in the equatorial zone of the epithelium elongate, the portion of each cell that is anterior to the nucleus grows forward under the undifferentiated epithelium, lying just beneath the anterior capsule; the posterior portion of each growing lens fiber simultaneously elongates backward beneath the posterior capsule. When the ends of similarly elongated cells meet each other close to either the anterior or posterior pole of the lens, both cells stop growing. New fiber cells are formed continually throughout life and older cells are displaced toward the center of the lens. As with tree rings, the youngest cells are at the lens periphery, the oldest at the center. A diagram of the adult lens is presented in Figure 10.6. Understandably, the lens center is highly compacted. It is referred to as the “lens nucleus.” The more superficial layers of younger fiber cells are called “lens cortex” (Fig. 10.6). An interesting feature of the differentiation of lens epithelium into lens fibers is that as the fibers are displaced to the interior of the lens, they reach a stage where they lose their nuclei, mitochondria, and other cytoplasmic organelles.21 Lack of organelles eliminates light scattering that otherwise would occur in particulate cytoplasm. In a mature lens most fibers lack organelles21.
![]() Figure 10.6. A diagrammatic section through the lens. (From Danysh BP, Duncan MK: The lens capsule. Exp Eye Res 88:151, 2009, with permission.) |
The zonules, which are suspensory ligaments that support the lens, develop from the neuroepithelium at approximately the 65-mm stage (3 months’ gestation), when the ciliary body and iris begin to develop from the optic vesicle. At the 110-mm stage (4 months’ gestation), the zonules are well developed, running from the inner surface of the ciliary body to the lens capsule. Still later, at the 240-mm stage (7 months’ gestation), the vascular hyaloid system within the vitreous regresses; it is absent by 8.5 months’ gestation, leaving the lens without a blood supply for the rest of its life.
The regulatory factors involved in lens development are complex and have been reviewed in detail elsewhere.22,23 Lens induction depends on the interaction between presumptive lens ectoderm and other tissues, particularly the retina. Transcription factors Pax-6, as well as Eya, Six, Sox, and Prox play an important role.22 Communication between developing tissues is a two-way street; signals emanating from the lens have a significant influence the development of other parts of the eye24 (Fig. 10.7). Fibroblast growth factors, insulinlike growth factors, and Wnt-signaling proteins all appear to play a critical role in regulation of lens cell proliferation and differentiation.23,25,26 Understandably, growth factor receptors play a key mechanistic role.27
General Structure of the Lens
The lens is a biconvex, transparent structure positioned in front of the vitreous body and behind the iris (Figs. 10.1 and 10.3). The lens of the young eye is generally colorless, but a yellowish to amber color develops with age. The front surface of the lens, facing the cornea, is bathed with aqueous humor which flows through the posterior chamber, through the pupil, and into the anterior chamber. The entire cellular mass of the lens (the epithelium, cortex, and nucleus) is contained within the elastic capsule (Fig. 10.6). The lens is held in place by suspensory ligaments (zonules) running from the ciliary body and inserting into the superficial lens capsule around the equator. The lens is also supported by its immediate apposition to the vitreous.
Cell division and, thus, growth of the lens continue throughout life; as new lens cells are formed, the older cells are displaced toward the interior of the lens. As a result, the lens grows in size and weight as we age (Fig. 10.8 and 10.9)..28 estimate is that the human lens thickness increases at about 0.02 mm per year.29,30 The anterior–posterior dimension of the lens in the newborn is 3.5 to 4.0 mm, reaching 4.75 to 5.0 mm at 90 years of age. The equatorial diameter in infants is about 6.5 mm, increasing to 9.0 with age. The radius of curvature of the anterior surface of the lens is in the range of 8 to 14 mm, whereas at the posterior surface, it is 4.5 to 7.5 mm. There is, however, variation in curvature with age and accommodation.
Capsule
The cell mass of the lens is completely enclosed by a transparent elastic acellular capsule. In the adult, the thickness of the lens capsule is approximately 13 μm at the anterior pole, but is considerably thinner (∼4 μm) at the posterior pole..31 The anterior capsule thickens with increasing age, but the thickness of the posterior capsule changes very little after birth. The dynamics of capsular growth have been discussed fully by Rafferty 32 and by Parmigiani and McAvoy.33 Viewed by light microscopy, the capsule material appears homogeneous. However, electron microscopy reveals a fibrillar structure with a more dense outer layer (the zonular lamella) into which the zonules penetrate 0.5 to 1 μm.34 The inner aspect of the capsule makes contact with the basal surfaces of the epithelial cells anteriorly (Fig. 10.10) and the elongated lens fiber posteriorly. Electron microscopic studies reveal a smooth contour on all surfaces except at the equator where the tension of the zonules produces irregularities.
The lens capsule serves as the basement membrane for lens cells. In this respect, there are differences between the posterior capsule which is the basement membrane for lens fibers and the anterior capsule which is the basement membrane for the epithelium.35 Cell–capsule interactions influence cell proliferation, differentiation, and fiber elongation. The capsule is composed mainly of type IV collagen combined with about 10% glycosaminoglycan.36,37 As with other basement membrane material, the lens capsule stains positive with periodic acid-Schiff (PAS) reagent in histologic section. The capsule is also easily digested by collagenase. Elegant autoradiographic studies by Young and Ocumpaugh38 demonstrated that the capsule is synthesized by the lens epithelium and superficial posterior lens fibers. Synthesis of capsular material appears to persist throughout life at the anterior of the lens but not at the posterior surface.33 The zonules, or suspensory ligaments, are anchored in the lens capsule. The zonules hold the lens in place and influence its shape by the degree of tension they exert. The zonules are structurally and biochemically similar to elastic microfibrils in other tissues.39,40,41 The capsule and zonules play an important yet passive role in the process of accommodation. Their degree of elasticity is important. As described above, the shape of the lens changes according to the degree of tension applied to the zonules by the ciliary muscle.
Epithelium
The lens epithelium exists as a monolayer situated beneath the capsule at the anterior surface of the lens (Fig. 10.10). The epithelial monolayer extends to the equatorial region of the lens but does not continue across the posterior surface. Thus, the outermost lens cells that face the aqueous humor are epithelial cells, whereas fibers are the outermost lens cells that face the vitreous humor. With the exception of cells at the equatorial zone, the epithelial cells are generally cuboidal, about 5.5 to 8 μm high and 7 to 11 μm wide..42 The lateral surfaces of these epithelial cells are richly interdigitated. Toward the equatorial zone, the epithelial cells tend to be more cylindrical. A small population of epithelial cells at the posterior of the equator, the germinative zone, represents the only group of cells in the mature lens that remains capable of mitosis. It is here that the cells divide and differentiate into new lens fiber cells.
The plasma membrane domains at the lateral and apical aspect of the epithelium have desmosomes for cell adhesion. The epithelial cell plasma membranes have also been shown to have gap junctions,43,44 which facilitate intercellular communication between the adjacent epithelial cells themselves and, less frequently, with the underlying lens fiber cells. (The role of gap junctions in the physiology of the lens will be discussed later.) All lens epithelial cells possess a nucleus together with granular cytoplasm, mitochondria, Golgi apparatus, ribosomes, rough endoplasmic reticulum, and numerous small filaments.42,45 Such intracellular organelles are consistent with the competent metabolic function of the epithelial cells. In addition to cell division, the epithelium is responsible for much of the active solute transport activity in the lens and also secretion of capsular material. Cytochemical studies of lens epithelium membranes have demonstrated the presence of sodium-potassium adenosine triphosphatase (Na,K-ATPase; the sodium pump enzyme) at the lateral and apical surface membranes.46,47 The epithelium contains a high specific activity of many other enzymes, including acid phosphatase and aldose reductase.48,49 Between adjacent cells, there appear to be tight junctions.50 In simple terms, lens epithelial cells have the characteristics of “normal” epithelial cells. The same is not true for lens fibers. Although they are differentiated epithelial cells, fibers are highly specialized and have several uncommon features.
Fiber Cells
The bulk of the lens is made up of tightly packed fiber cells (Fig. 10.11). The most superficial, newly made, lens fibers contain organelles and a nucleus similar to those of the anterior epithelial cells.42,45,49 However, these structures are not retained. As the cells age and are displaced farther toward the center of the lens, a stage is reached where the cell nucleus fragments and disappears completely, together with other organelles. The ability to get rid of these intracellular structures is a highly coordinated process that seems to be programmed into the fiber cell (Fig. 10.12).21 The fully differentiated, mature lens fiber has no nucleus, mitochondria, or endoplasmic reticulum. The nonparticulate cytoplasm of the mature fiber cells is a specialization that contributes to the transparency of the lens.
The newly formed elongating lens cells have a hexagonal cross section (Fig. 10.13) that they retain throughout life. Fibers are enormously long, but thin, hence their name. Within the cortical region of the lens, the fiber cells are 8 to 12 mm long, 7 μm wide, and 4.5 μm thick..42 The lens fibers are very densely packed, forming a highly structured, honeycomblike array with an intercellular distance of only about 20 nm. The intercellular dimension is small compared with the wavelength of visible light and this, together with the high degree of order, minimizes light scatter and favors transparency. A recently formed lens fiber has its center approximately at the equator, whereas its two ends project forward and backward to the anterior and posterior poles of the lens. If the system were perfectly symmetric, all the fibers would end at the same location at either pole of the lens; this does not happen, perhaps because it would detract from the optical properties of the optical axis of the lens. Instead, the fiber ends meet in complex patterns called lens sutures. The human embryonic lens has Y-shaped sutures, but in the adult lens there is a more complicated four-pointed star arrangement.32
Adjacent lens fiber cells lying most superficially show some moderate interdigitations, but deeper lens fibers have distinct interlocking processes resembling ball-and-socket joints.32,51 Moving further into the nuclear region, the lens fiber cells retain their hexagonal shape yet the interlocking processes are replaced by ridges. The purpose of these interlocking mechanisms between lens fiber cells may be stabilization of the packing arrangement to prevent slippage of cells against each other when the lens changes shape during the process of accommodation.
Junctions Between Cells
Because terminally differentiated lens fiber cells lack a nucleus, mitochondria, and other organelles, they are incapable of existing in isolation. Their survival depends on the ability of the lens cell mass to function similar to a syncytium, with the epithelium and the nucleated fiber cells at the lens surface to support the non-nucleated fiber cells underneath. This is made possible by gap junction channels between fiber cells (Fig. 10.14) and possibly also by the occasional fusion of adjacent fiber cells. By means of these specializations, low-molecular-weight solutes can pass from cell to cell (Fig. 10.15). Gap junctions also connect adjacent cells in the anterior monolayer of epithelium, but junctions between epithelial cells and fibers are sparse.52,53 Gap junctions are formed when hemichannels in adjacent cells dock, creating a pathway for the passage of ions and low-molecular-weight solutes. Each hemichannel is an assembly of connexons that are formed by specific connexin proteins. More than a dozen connexins are known to exist, coded by different genes. Connexin-46 (Cx46) and connexin-50 (Cx50) are found in mammalian lens fibers.54,55 The gap junctions between lens fibers are different from those between lens epithelial cells in which connexin-43 (Cx43) is predominant.56 The importance of gap junctions is reinforced by the finding that targeted mutation of either Cx46 or Cx50 in mice results in cataract.55,57 In humans, mutations in Cx46 and Cx50 genes have been associated with certain congenital cataracts.58,59 Curiously, a water channel protein, aquaporin-0 (AQP-0), might play a role in junction formation between lens fibers.60 Both connexons and AQP-0 tend to organize into latticework microdomains. Although AQP-0 appears responsible for thin junctions, atomic force microscopy reveals an association between patches of connexons and AQP-0 (Fig. 10.16).61 Together, the two junctions might provide for adhesion and a pathway for diffusion of substances from fiber to fiber. Diffusion in along the clefts of extracellular space between fibers may be restricted by another junction protein, MP-20, which is related to the claudin family.62
The distribution of gap junctions does not appear to be spatially uniform across the fiber cell mass and the extent of functional cell–cell coupling is highest at the equator and quite low at the anterior and posterior poles.53 Fiber cells located within 400 μm of the lens surface are able to uncouple reversibly in response to stimuli, such as a change of pH; the fiber cell connexons can apparently regulate their conductance. More deeply located, older fibers are not capable of uncoupling and this may stem from post-translational modification of the connexin proteins or the absence of essential cofactors. There could, however, be other pathways that couple deeply located fibers. It has been found that cell-to-cell fusion occurs in the lens core63 (Fig. 17).
![]() Figure 10.17. A three-dimensional representation showing the structure of two neighboring fiber cells in the lens core illustrating that, although the fibers are present as discrete, separate cells throughout most of their length, they are fused in zone b. Such fusion of adjacent fiber cells is likely to permit cell-to-cell movement of fairly large molecules as illustrated in Figure 1.13. (From Shestopalov VI, Bassnett S: Expression of autofluorescent proteins reveals a novel protein permeable pathway between cells in the lens core. J Cell Sci 113:1913, 2000, with permission.) |
Water
The lens is unusual in that it has a low water content (65%) and an extraordinarily high protein content (33%). Almost all of the lens dry weight is protein. Low water content and high concentration of refractile protein are essential for the optimal optical function of the lens. The composition enables the lens to have a refractive index considerably greater than its fluid environment and yet remain sufficiently hydrated to be deformable during the process of accommodation. Lipids, inorganic ions, organic phosphate, nucleic acids, and various metabolites comprise only about 2% of the total lens solids (Table 10.1). It should be noted, however, that the composition of the lens varies with aging. Furthermore, a number of dramatic changes in lens composition accompany cataract.
TABLE 10.1. Lens Composition | ||||||||||||||||||||||||||
---|---|---|---|---|---|---|---|---|---|---|---|---|---|---|---|---|---|---|---|---|---|---|---|---|---|---|
|
The low water content of the lens partly reflects the unique protein composition of fiber cell cytoplasm. It also reflects a lack of extracellular space. Although the adult human lens capsule is about 80% water,64 the cortex contains 68.6% water and the nucleus 63.4% water.65 The normal human lens shows no significant alteration in hydration with age.65,66 Not all the water in the lens is freely diffusible. A fraction is involved in protein hydration and is thus considered “bound.” Earlier evidence for different fractions of lens water was obtained using flux studies with radioactively labeled water. Nuclear magnetic resonance (NMR) studies suggest that about half of the lens water is physically associated with lens protein.67 Because the fibers are packed tightly, very little of the lens water is located in the spaces between the cells. The extracellular space of the lens has been measured by determining the distribution of tracer substances (e.g., inulin) that do not penetrate the lens cell membranes. In the rabbit lens, the inulin space has been reported to be about 5%.64,68 Nearly a fourth of the measured extracellular space is located in the capsule.64
Lens hydration can be significantly higher in certain forms of cataract. Precise regulation of lens hydration is critical for the maintenance of lens transparency. Cell swelling, cell shrinkage, or enlargement of the extracellular spaces results in disruption of the highly ordered arrangement of lens fibers and this causes light scatter. Fischbarg et al.69 suggest that the lens epithelium is able to transport water inward to the fiber mass. Water may be shifted out of the lens at the equator. The expression of specialized water channel proteins, aquaporins, might enable the lens to perform these functions70.
Protein
The lens is a highly specialized organ and it should come as no surprise that lens cells have a distinctive pattern of protein expression. Lens fiber cytoplasm is rich in crystallins and fiber membranes are rich in aquaporin-0. In the human lens, the major proteins are α, β, and γ crystallin. In reptilian, avian, and invertebrate species there are other crystallins (Table 10.2). α-crystallin accounts for as much as 40% of total lens protein. α-crystallin is not a single protein but a complex or aggregate of 30 to 40 copies of αA-crystallin and αB-crystallin in roughly a 3:1 molar ratio. The αA- and αBβ-crystallin polypeptides have a molecular mass of approximately 20 kDa. At the time of synthesis, the α-crystallin complex has a molecular weight of about 7 × 105 Da. With aging, larger aggregates of α-crystallin are formed with molecular weights as high as 50 × 106 Da; these aggregates of αcrystallin become water insoluble and have a tendency to bind to the plasma membrane of fiber cells.71,72 This might contribute to a decrease in transparency.
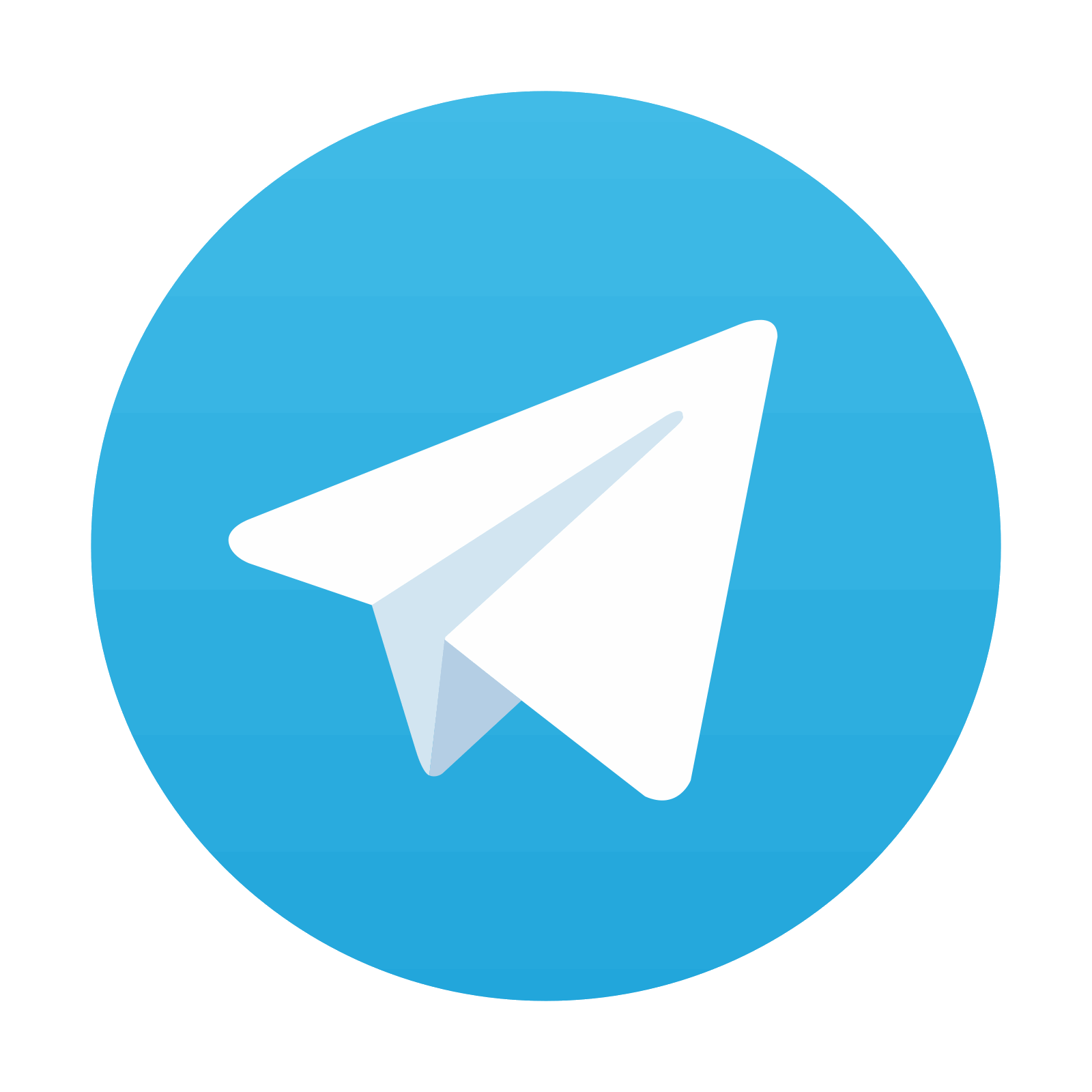
Stay updated, free articles. Join our Telegram channel
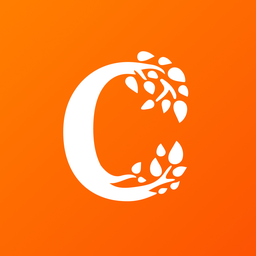
Full access? Get Clinical Tree
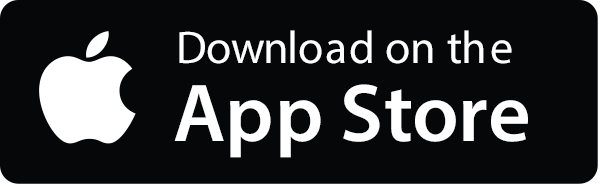
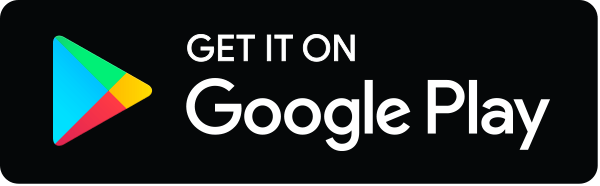