(14.1)

(14.2)
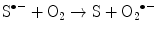
(14.3)
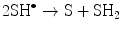
(14.4)

(14.5)

(14.6)
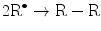
(14.7)

(14.8)
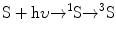
(14.9)
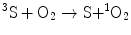
(14.10)
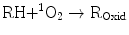
(14.11)
14.2 Dye-Sensitized Capacity of Advanced Glycation End Products
In the literature, there is an extensive list of low molecular weight compounds that changes their relative concentration in the human eye lens during aging and in nondiabetic cataracts [26, 37–40]. These compounds have been assigned different possible roles based on whether they act as sensitizers and prooxidants, antioxidants or benign filters that protect the posterior parts of the eye from light damage; some compounds still have an uncertain role [25, 41].
In this section, we discuss the photochemical mechanisms that take place during the irradiation of amino acid models of advanced glycation end products and their effects on key molecules that are targets of oxidative stress in the eye lens.
The generation of advanced glycation end products (AGEs) in lens proteins has been proposed as a major protein modification contributing to the formation of age-onset and diabetic cataracts [20, 26, 42–46]. In the eye lens, the formation of AGEs can occur with either glucose (important in diabetic patients with high sugar levels) or ascorbic acid oxidation products [45–48]. The degradation of glucose and ascorbic acid can produce intermediaries, such as threosone and 3-deoxythreosone, that possesses carbonyl groups that can react with proteins, giving rise to AGEs [49, 50]. Evidence of the presence of tetroses has been reported in the aqueous humor of cataractous subjects [51] as well in the water-soluble and -insoluble fractions of lens proteins from cataractous patients [52], indicating the relevance of the nonenzymatic degradation of glucose and ascorbic acid.
Most of the known AGEs can be formed from nonenzymatic degradation intermediates, as another route to the well-known cellular metabolic pathways. These intermediates generate electrophilic compounds, such as methylglyoxal, which can also give rise to AGEs [53–55]. The generation of AGEs via nonenzymatic reactions is especially important in mature eye lens fibers, where the contribution to AGEs generated through metabolic pathways is expected to be negligible due to the absence of organelles and a reduced or absent glucose metabolic activity [56].
In proteins, glycation process is initiated by a nucleophilic attack of sulfhydryl or amino groups to carbonyl groups of carbohydrates or their degradation products, which leads to the formation of a Schiff’s base, followed by an Amadori rearrangement (aldoses) or Heyns rearrangement (ketoses). The generation of intermediary compounds containing new carbonyl groups allows the reaction with another nucleophilic group, initiating multistep transformations that ultimately lead to a variety of structures known generically as AGEs. The generation of these compounds is accompanied by the simultaneous appearance of a brown coloration with absorption bands in the UVA and visible regions of the spectra. Some of these chromophores, which also have fluorophore properties (λ em = 450 nm), have been probed as sensitizers when they are irradiated in the UVA region [23, 57]. However, there are no comparative studies concerning their photosensitizing activities when the irradiations are performed at low oxygen pressure and using different wavelengths to investigate the probable simultaneous occurrence of two or more sensitizing processes in the reaction mixture. These conditions are very important because they simulate the physiology of a tissue, such as the eye lens, that has very low or no protein turnover, is not vascularized, is exposed to the direct effect of light and whose proteins are exposed to glycation for a long period of time, depending on the age of the subject. Research concerning UVA-absorbing compounds in the eye lens and their photochemical properties has been a subject of increasing interest [12, 16, 26, 58, 59]. Under aerobic conditions, excited states of AGEs are deactivated, generating singlet oxygen as the predominant specie [60] and overcoming the generation of superoxide radical anion [61] and hydrogen peroxide [62]. Nonetheless, assessments of the actual photosensitizing potential of AGEs did not take into account the low oxygen concentration in this tissue, which can have a direct influence on the prevalence of the Type I photosensitizing mechanism. Consequently, these studies overlooked the reactive species produced through this pathway. In recent years, new studies have been performed in vitro, using models of AGE as photosensitizers and taking into consideration the low oxygen pressure of the lens. The purpose of these studies, whose main results are shown below, was the comparative evaluation of the photosensitizing activity of AGEs obtained in vitro through the incubation of ascorbate (ASC), glucose (Glc), or threose (Threo) with lysine (Lys), employing the characteristic low oxygen pressure present in the eye lens [63]. The essential amino acid tryptophan (Trp) and vitamin C were used as targets to evaluate the efficiencies of the photo-processes which were performed with poly- and monochromatic light at 5 % O2.
Table 14.1 shows the quantum yields for Trp decomposition when this amino acid was irradiated with monochromatic light (344 or 367 nm) in the presence of the different sensitizers. It was observed that the quantum yields are from 2.8- to 5.7-times lower at 367 nm than at 344 nm; this finding confirms the existence of at least two species with different sensitizing capacities in the samples. When the emission properties of the chromophores and their photosensitizing activities during Trp decomposition were compared, it was observed that at 344 nm (Fig. 14.1a) and 367 nm (Fig. 14.1☻b), Trp decomposition quantum yields increased when the fluorescence emission yields of the sensitizers decreased. This inverse correlation can be mainly explained by considering that photosensitization is a triplet process and its quantum yield is inversely related to the fluorescence yield.
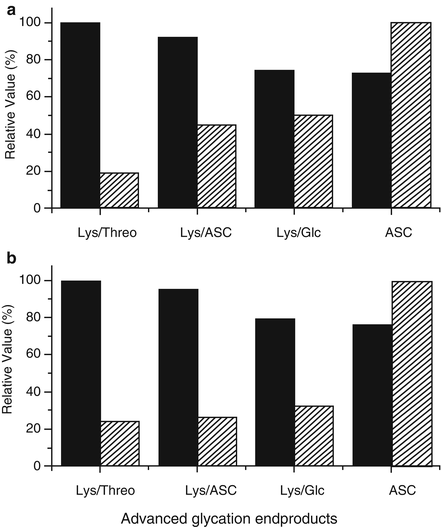
Table 14.1
Initial quantum yields for Trp sensitized decomposition at 344 and 367 nm in the presence of the different chromophores (absorbance of 0.2 at 365 nm)
Sensitizers | ϕ 344 × 103 | ϕ 367 × 103 |
---|---|---|
ASC | 5.41 ± 0.98 | 1.23 ± 0.01 |
Lys/Glc | 1.72 ± 0.31 | 0.61 ± 0.06 |
Lys/ASC | 1.38 ± 0.28 | 0.55 ± 0.11 |
Lys/Threo | 1.31 ± 0.66 | 0.23 ± 0.18 |
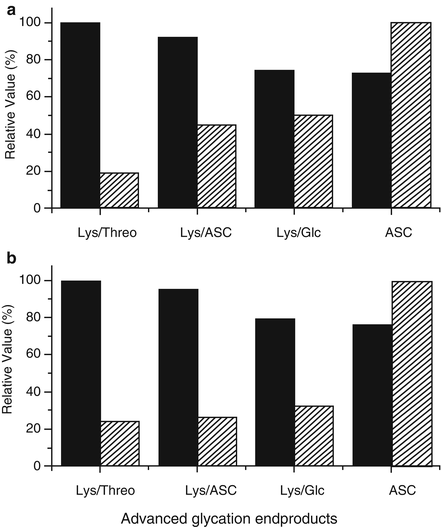
Fig. 14.1
Relative Trp photodecomposition quantum yields (striped columns) and emission fluorescence efficiencies (black columns) at 344 (a) and 367 nm (b). One Hundred percent was assigned to the highest value of each set of data. Fluorescence emission efficiencies were expressed as relative emission intensities at the corresponding wavelengths divided by the fraction of absorbed energy (1 − Tλ). Reproduced from Photochem. Photobiol. 2007, 83: 563-569 with permission from Wiley
Taking into account that physiological ASC concentrations in the eye lens can be extremely high (between 1 and 2 mM) [64] and that its decomposition promotes the generation of colored compounds and also glycating agents, this vitamin was used as a target of the photo-processes mediated by amino acid models of AGEs studied in this work. The obtained ASC decomposition quantum yields mediated by AGE are significantly higher (ϕ 367 × 103: 256 ± 10 for ASC, 110 ± 8 for Lys/Glc, 67.9 ± 3.9 for Lys/ASC and 26.2 ± 2.8 for Lys/Threo) than those obtained for Trp decomposition (see Table 14.1), and the efficiency on the photodegradation was also dependent on the type of carbohydrate used as a precursor of AGE synthesis. The presence of copper ions in the reaction media can contribute to an active propagation of ASC oxidation. To avoid this effect, the experiments were carried out in the presence of the metal chelator DTPA.
Importantly, the efficient photosensitizing activity of the degradation products of ASC can autosensitize its own decomposition resulting in the complete depletion of this antioxidant. Trp in free form or as a constituent of proteins is normally one of the main targets of photosensitizing processes mediated by AGEs [29]. It has been demonstrated that the AGE-photosensitized Trp oxidation rate increases with AGE concentration and is optimal at 5 % oxygen [29]. By contrast, for ASC, the photooxidation rate increases with oxygen concentration. As ASC is more rapidly photo-degraded than Trp, the antioxidant competitively protects Trp from oxidation up to 1 mM, although not absolutely [29]. There is no exact information in reference to the chemical nature of the compounds responsible for this coloration, but the main products of ASC degradation are well documented [49, 52, 65–68].
The mechanistic aspects of the photo-processes mediated by a model of AGEs, obtained by the incubation of Nα-acetyl-l-lysine, Nα-acetyl-l-arginine and d-glucose, have also been analyzed [69]. To investigate the photosensitizing mechanism of AGEs, Trp was used as a model target and the photodecomposition quantum yields at 367 nm of this amino acid were determined at different oxygen concentrations. FeCy, a known electron-scavenger [70], was used with the aim of interfering with the electron-transfer mechanism, whereas deuterium oxide buffer was used to enhance singlet oxygen-mediated photodamage.
The results shown in Table 14.2 support a combined Type I–Type II mechanism for all oxygen concentrations, with Type I and Type II favored at low and high oxygen concentrations, respectively. The fraction of Type I (fType I) and Type II (fType II) mechanisms that contributes to Trp photodecomposition depends on both Trp and oxygen concentration, and the rate constants of the reactions between triplet AGEs and Trp or O2. This relationship is expressed by the equations below:
![$$ {\mathrm{f}}_{\mathrm{TypeI}}={k}_{\mathrm{et}}\left[\mathrm{T}\mathrm{r}\mathrm{p}\right]/\left({k}_{\mathrm{ic}}+{k}_{\mathrm{P}}+{k}_{\mathrm{et}}\left[\mathrm{T}\mathrm{r}\mathrm{p}\right]+{k}_{\mathrm{q}}\left[{\mathrm{O}}_2\right]\right), $$](/wp-content/uploads/2017/03/A314033_1_En_14_Chapter_Equ12.gif)
![$$ {\mathrm{f}}_{\mathrm{TypeII}}={k}_{\mathrm{q}}\left[{\mathrm{O}}_2\right]/\left({k}_{\mathrm{ic}}+{k}_{\mathrm{P}}+{k}_{\mathrm{et}}\left[\mathrm{T}\mathrm{r}\mathrm{p}\right]+{k}_{\mathrm{q}}\left[{\mathrm{O}}_2\right]\right), $$](/wp-content/uploads/2017/03/A314033_1_En_14_Chapter_Equ13.gif)
where k et, k ic, k P, and k q correspond to the rate constants of the electron transfer between triplet AGE and Trp; internal conversion; phosphorescence emission; and the quenching of triplet AGE by oxygen, respectively.
Table 14.2
Quantum yields for AGE-sensitized Trp decomposition at 367 nm under different oxygen pressures
Trp photodecomposition quantum yields (ϕ 367 × 103) | |||
---|---|---|---|
O2 (%) | Control | Added FeCya | D2O bufferb |
5 | 0.55 | 0.28 | 1.44 |
20 | 0.35 | 0.29 | 1.74 |
100 | 0.49 | 0.38 | 2.42 |
![$$ {\mathrm{f}}_{\mathrm{TypeI}}={k}_{\mathrm{et}}\left[\mathrm{T}\mathrm{r}\mathrm{p}\right]/\left({k}_{\mathrm{ic}}+{k}_{\mathrm{P}}+{k}_{\mathrm{et}}\left[\mathrm{T}\mathrm{r}\mathrm{p}\right]+{k}_{\mathrm{q}}\left[{\mathrm{O}}_2\right]\right), $$](/wp-content/uploads/2017/03/A314033_1_En_14_Chapter_Equ12.gif)
(14.12)
![$$ {\mathrm{f}}_{\mathrm{TypeII}}={k}_{\mathrm{q}}\left[{\mathrm{O}}_2\right]/\left({k}_{\mathrm{ic}}+{k}_{\mathrm{P}}+{k}_{\mathrm{et}}\left[\mathrm{T}\mathrm{r}\mathrm{p}\right]+{k}_{\mathrm{q}}\left[{\mathrm{O}}_2\right]\right), $$](/wp-content/uploads/2017/03/A314033_1_En_14_Chapter_Equ13.gif)
(14.13)
Given that the Trp concentration was the same in all the experiments, the contribution of Type I and Type II photo-processes depends solely on the oxygen concentration and the values for the rate constants of the processes.
At 5 % O2, the Type I photosensitizing mechanism is predominant because the presence of FeCy considerably drops the quantum yield to 51 % with respect to the control. A similar result was reported previously for the predominantly Type I sensitizer, riboflavin [28]. In the same conditions, D2O increases the damage 2.6 times, which is low if we consider that deuterium oxide prolongs the lifetime of singlet oxygen approximately 10–17 times compared to water [71]. This result indicates a small contribution of singlet oxygen at low oxygen concentration.
At 20 % O2, the quantum yield drops to 64 % with respect to 5 % O2. This result is consistent with the finding that AGEs acts preferentially as Type I sensitizers. Therefore, the fourfold increase in the oxygen concentration disfavors the interaction between the triplet sensitizer and tryptophan, which is reflected in a lower damage to the target. The presence of FeCy does not inhibit the process to a great extent (down to 80 % of the control), which agrees with the fact that at 20 % oxygen, the Type I mechanism is disfavored. A high oxygen concentration favors the quenching of the triplet state of the sensitizer by ground state oxygen in a Type II mechanism that generates singlet oxygen, which is observed as a fivefold increase in the quantum yield when the reaction is performed in deuterium oxide buffer.
At 100 % O2, the quantum yield rises again to 89 % of the value observed at 5 % O2. This result was surprising because for a Type I sensitizer, an even greater decrease in the quantum yield was expected compared to the value at 20 % O2. A plausible explanation for this behavior is that at 100 % O2, the increased amount of singlet oxygen generated in this condition, compensates for the loss in a Type I mechanism, thereby balancing the total efficiency of the process. The influence of FeCy and D2O in the quantum yield shows the same behavior as that observed at 20 % O2 and is consistent with the predominance of the Type II mechanism at high oxygen concentrations. Singlet oxygen concentrations from 1.2 to 2.7 μmol/mL have been reported for purified threose AGE compounds irradiated with UVA [23].
Notwithstanding the fact that an enhancement of the photodamage in the presence of deuterium oxide was observed for all oxygen concentrations, supporting the participation of the Type II mechanism, the increase was small compared to the expected enhancement of the lifetime of singlet oxygen. The ratio between the quantum yields in D2O and in H2O is 2.62 at 5 % O2, which is low compared to that for known Type II sensitizers such as methylene blue, where a ratio of 8.29 in oxygen-saturated solutions was observed [72]. In the same study, a ratio of 4.99 was observed for riboflavin in aerated solutions, which agrees well with the values of 4.97 and 4.94 observed for 20 % and 100 % oxygen concentrations, respectively, that were found for AGE in our conditions. These data indicate that Type I photo-processes are the principal mechanism by which AGEs induce photodamage under low oxygen conditions, which corresponds with the in vivo condition in the eye lens.
14.3 Autosensitized Oxidation of Colored and Glycated Bovine Lens Proteins Exposed to UVA-Visible Light at Low Oxygen Concentrations [73]
Photooxidative damage produced by AGEs generated during aging has been proposed as a possible mechanism of cataract formation [3, 23, 25, 26, 57]. In this part of the chapter, the photosensitizing ability of the chromophores generated by the incubation of bovine lens proteins (BLPs) with ASC will be analyzed, taking into consideration the actual low oxygen concentration present in the eye lens [74]. As lenticular AGEs are believed to mainly derive from the reaction between crystallins and the oxidation products of ASC [16], ASC was chosen to induce the glycation reaction and the concomitant production of the colored compounds required to perform photochemical studies of AGEs bound to proteins.
Glycated samples of lens proteins (10 mg/mL) were incubated for 3 and 6 days in the presence of 30 mM ASC and 5 μM CuSO4, respectively, resulting in an increase in the absorbance in the UVA-visible region that is associated with the generation of AGEs (Fig. 14.2a). These compounds originate from the initial reaction between amino groups of basic amino acid residues (e.g., the ε-amino groups of Lys residues) and carbonyl groups arising from the decomposition products of ASC. This reaction leads to the formation of a Schiff’s base, followed by an Amadori rearrangement. The generation of intermediary compounds containing new carbonyl groups allows the reaction with another amino group, initiating the multistep transformation and ultimately leading to a variety of structures known as advanced glycation end products (AGEs). The ASC-glycated proteins exhibited a 57 % loss in their amino group content at the end of 6 days (Fig. 14.2c), an amount that is more than sufficient to explain the presence of 27 % cross-linked proteins (Fig. 14.2b). The majority of the generated cross-links corresponds to dimeric forms, which derive from the formation of these AGE-chromophores in which two amino groups from different protein subunits participate. This fact will determine the protein residues that will be exposed to the reactive species generated during the photosensitization processes mediated by these covalently bonded chromophores.
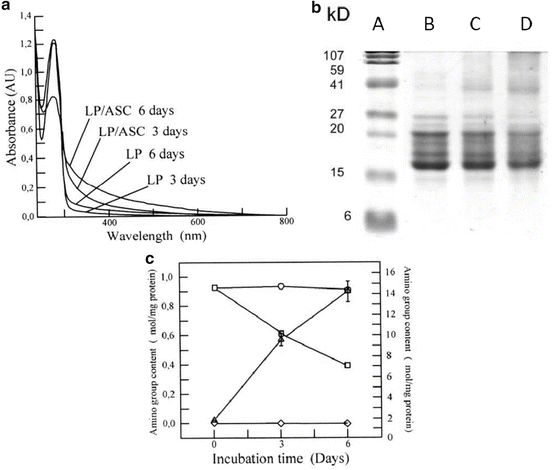
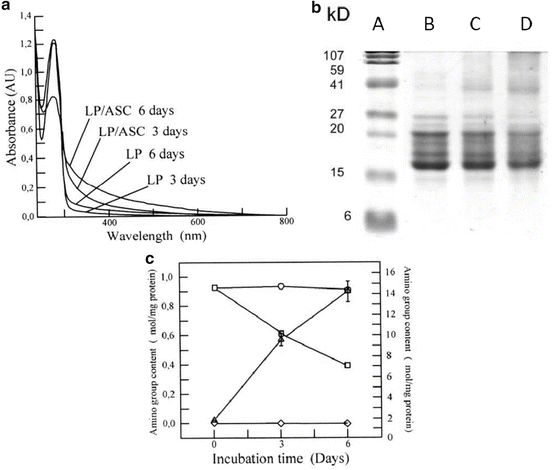
Fig. 14.2
Glycation of bovine lens proteins (LP) with ascorbic acid (ASC). (a) Shows the UV-visible spectra and time course production of advanced glycation end products. Samples were dialyzed and the spectra were recorded at a concentration of 1 mg/mL. (b) Shows the changes in the SDS-PAGE patterns of the samples. Lanes a–d correspond to the MW standard, 1, 3 and 6 days of incubation, respectively. (c) Shows the loss in amino group content (open square) and the rise in carbonyl groups (filled triangle) due to the glycoxidation processes. Controls for amino group loss (open circle) and carbonyl group formation (filled diamond) were performed by incubating the protein in the absence of ASC. Reproduced from Photochem. Photobiol Sci. 2008, 7: 718-724 by permission of The Royal Society of Chemistry (RSC) for the European Society for Photobiology, the European Photochemistry Association, and the RSC
Protein carbonyl groups were also detected in parallel with the formation of AGEs and the decrease in amino group content (Fig. 14.2c). Under the experimental conditions employed in this work, carbonyl groups could be introduced into proteins by a variety of oxidative processes. One of these processes is related to the prooxidative capacity of ASC in the presence of trace amounts of Cu2+, especially when the antioxidant reaches low concentrations and is not able to trap the reactive oxygen species (ROS) generated by the reoxidation of the reduced transition metal [75]. It is possible that this process occurs due to oxidative Maillard reactions catalyzed by the copper present in the incubation medium. In this case, the Amadori products undergo a tautomerization process, which gives rise to a protein enediol. This reaction marks the start of glycoxidation, in which the enediol is oxidized to a protein dicarbonyl in a reaction catalyzed by the transition metal and with the participation of molecular oxygen, which is reduced to a superoxide radical anion and finally is transformed to the hydroxyl radical via hydrogen peroxide in a new step that requires the presence of metals [76, 77]. The different ROS generated during the glycoxidation process are responsible for the oxidation that affects the side chains of reactive amino acids, such as His, Trp, or Tyr [78, 79]. This phenomenon was experimentally observed as a decrease in the fluorescence intensity of the Trp residues in the glycated proteins. Notwithstanding the fact that the precursors of AGEs include different intermediates that contain carbonyl groups, such as Amadori or Heyns products, it has been reported previously that neither of these products contributes to the 2,4-dinitrophenylhydrazine DNPH reaction because these groups are rapidly lost in a first-order reaction [80]. Consequently, the observed increase in the carbonyl content found during the incubation of lens proteins with ASC must be mostly attributed to oxidative processes mediated by ROS.
It has been demonstrated that low level CL is emitted after human serum albumin oxidation mediated by AGEs and ROS formation [63]. This CL lasts for several minutes after the end of the photolysis and is due to the formation of excited states generated in the decomposition of peroxides and/or hydroperoxides formed during the oxidation of Trp residues inside the proteins [81]. The CL observed after the exposure of ASC-glycated lens proteins to visible light at low oxygen pressure indicates the occurrence of autosensitized photo-processes inside the proteins mediated by the covalently bonded AGE-chromophores (Fig. 14.3). As a consequence of the process, some amino acid residues become targets of this oxidation. The high sensitivity of this method allows the observation of CL even when the samples are illuminated with visible light, notwithstanding the fact that the absorption properties of the irradiated samples are very low in this region. This result is especially relevant because it indicates that even visible light can contribute to the enhanced photo-processes found in aged lenses. Native proteins incubated in the absence of ASC were insensitive to the action of visible light.
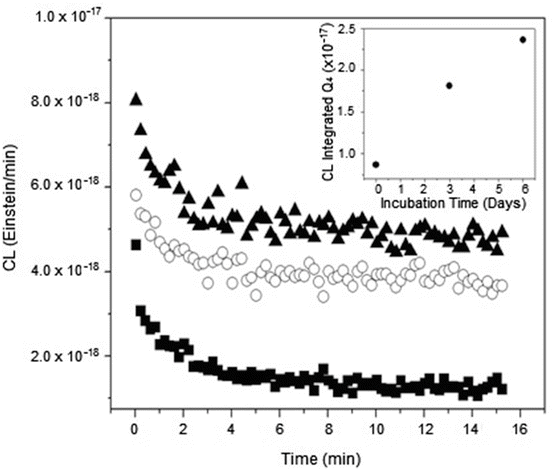
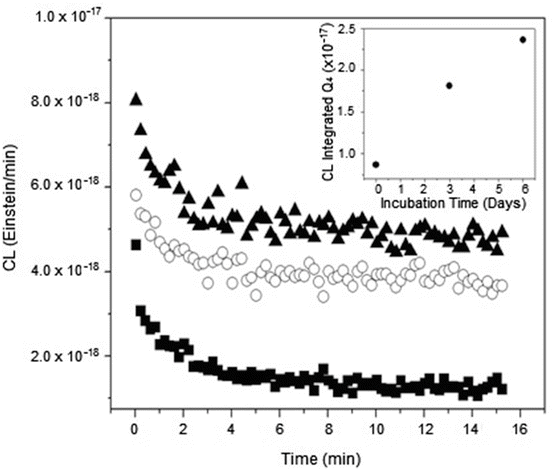
Fig. 14.3
Time profile of the chemiluminescence (CL) observed after the 5 min irradiation of bovine lens proteins glycated with ascorbic acid under 5 % O2 pressure. CL measurement was performed after 30 s at 25 °C. The insert shows the correlation between the CL integrated along the first 20 min of decay and the incubation time. Symbols: (filled square) 6 days incubated native proteins in the absence of ASC, (open circle) 3 days glycation and (filled triangle) 6 days glycation. Reproduced from Photochem. Photobiol Sci. 2008, 7: 718-724 by permission of The Royal Society of Chemistry (RSC) for the European Society for Photobiology, the European Photochemistry Association, and the RSC
Previous experiments performed with small molecular weight AGEs prepared with amino acids instead of the protein showed that the efficiency of these compounds at low oxygen pressure during Trp oxidation is higher than that found in an aerobic atmosphere [29], indicating the prevalence of Type I sensitized photo-processes. When lens proteins were irradiated with UVA-visible light in the presence of low molecular weight AGEs, the formation of cross-linking was observed (Fig. 14.4), indicating that Type I processes involving an electron transfer between the reactants took place, giving rise to radical intermediaries that are responsible for the aggregation processes. The absence of modifications in the electrophoretic patterns of the glycated and subsequently irradiated proteins (Fig. 14.5) indicates that radical species generated during the electron transfer of a Type I mechanism are not able to give rise to the intermolecular cross-linking observed when the same experiments were performed using amino acid models of AGE-chromophores as sensitizers (Fig. 14.4). In the case of the protein-bound AGEs, the sensitizing damage is restricted to the area near the sensitizer. The fact that the main increase in the carbonyl content after the irradiation of the glycated lens proteins was found in the cross-linked fractions (Fig. 14.6), which are those that contain most of the AGE-chromophores, suggests that the damage is located in the vicinity where the sensitizers are positioned. Nonetheless, considering that the protein concentration can be assumed to be as high as 300 mg/mL in the lens of the eye [67], the possibility of intermolecular reactions sensitized by the AGEs cannot be discarded.
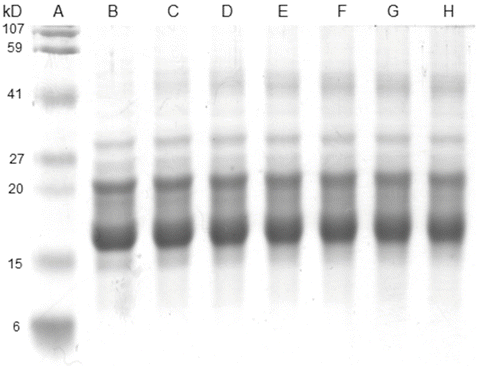
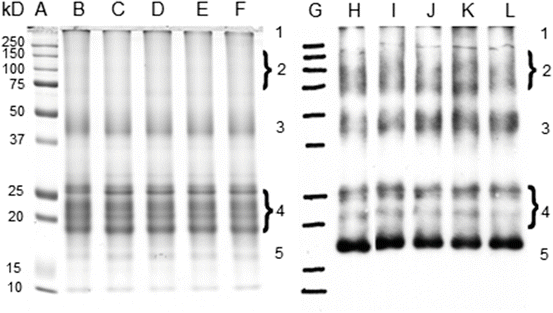
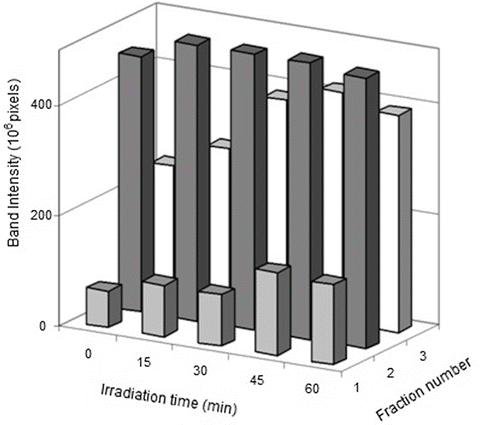
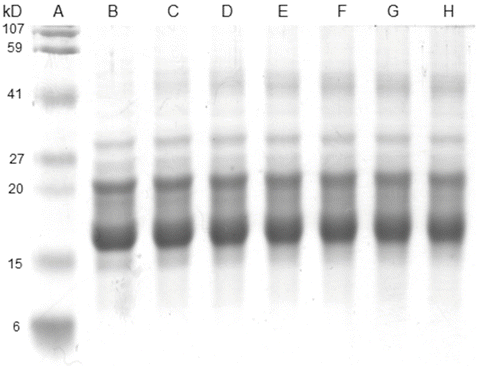
Fig. 14.4
SDS-PAGE of bovine lens proteins previously irradiated with UVA-visible light in the presence of small molecular weight AGEs generated from the incubation of ascorbic acid with N-acetyl-lysine and N-acetyl-arginine. Lanes a–h correspond to MW standard, 0, 10, 20, 30, 40, 50 and 60 min irradiated samples. Reproduced from Photochem. Photobiol Sci. 2008, 7: 718-724 by permission of The Royal Society of Chemistry (RSC) for the European Society for Photobiology, the European Photochemistry Association, and the RSC
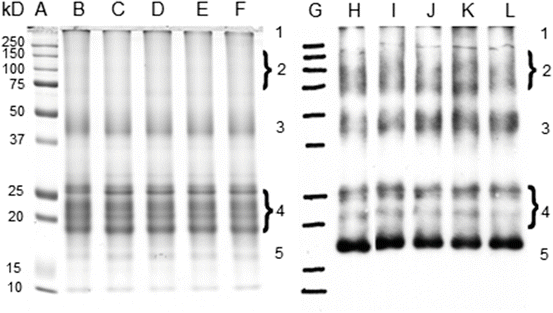
Fig. 14.5
Irradiation of 6-days glycated lens proteins with UVA-visible light at 5 % O2 pressure. Lanes a–f show the SDS-PAGE analysis of the irradiated samples corresponding to the MW standard, 0, 15, 30, 45 and 60 min of irradiation, respectively. Lanes g–l show the Oxyblot analysis of the same samples. Reproduced from Photochem. Photobiol Sci. 2008, 7: 718-724 by permission of The Royal Society of Chemistry (RSC) for the European Society for Photobiology, the European Photochemistry Association, and the RSC
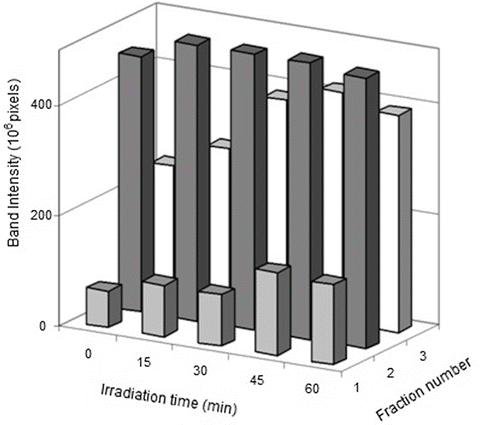
Fig. 14.6
Increase in the carbonyl content of previously glycated proteins with the irradiation time. The intensity of the bands was measured with Image Master 1D Software. No modifications were observed on bands 4 and 5 of Fig. 14.5 and, therefore, they were not included in this figure. Reproduced from Photochem. Photobiol Sci. 2008, 7: 718-724 by permission of The Royal Society of Chemistry (RSC) for the European Society for Photobiology, the European Photochemistry Association, and the RSC
The production of singlet oxygen when protein preparations from aged lenses were irradiated with UVA light has also been reported [57, 82]. The actual oxygen content in the lens is a topic that is still under discussion. Values of 28 mmHg in the cortex of the rabbit lens [83] and 2.5 mmHg for the bovine nucleus [33], and even the possibility of an essentially anaerobic lens [84] have been reported. In this context, the Type I sensitizing mechanism, which in its first step does not require the presence of molecular oxygen and involves the direct interaction between the sensitizer in triplet state and an adequate quencher, could play a very important role in the photosensitized events that occur in vivo in the eye lens. In addition to this finding, Ortwerth et al. reported the generation of hydrogen peroxide [62] and superoxide anion [61] as a result of the UVA irradiation of human lens proteins. This reactive species can derive from the Type I mechanism. Sensitized photochemical reactions mediated by AGEs are characterized by low quantum efficiency, but this fact is contrasted by the important contribution of these chromophores to the absorption of the incident radiation impinging on the lens [30], including the radiation of the visible region.
As a final remark, these results have demonstrated that the covalently bonded chromophores located in the cross-linked and glycated lens proteins can induce photooxidative damage when the samples are exposed to UVA-visible light under the low oxygen pressure present in the eye lens. Nonetheless, this damage is restricted to the area close to the chromophores and cannot explain the protein cross-linking observed during aging and in patients with cataracts (see Scheme 14.1).
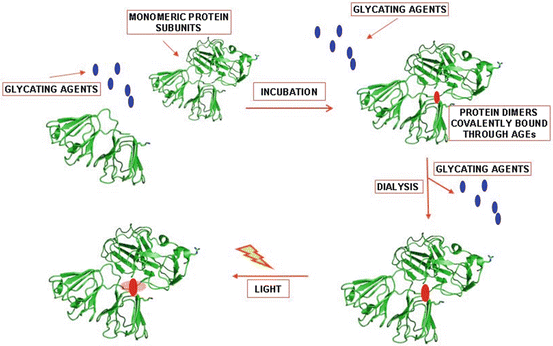
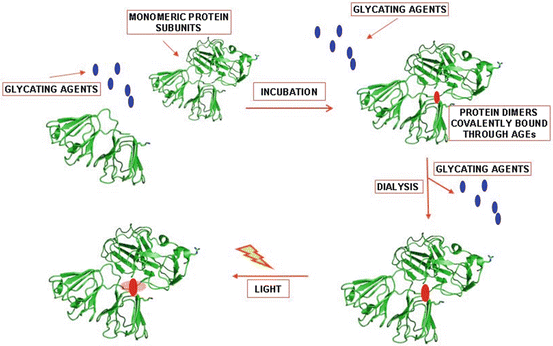
Scheme 14.1
Effect of UVA-visible light on bovine eye lens proteins, previously cross-linked in the absence of light, after incubation in the presence of ascorbate (ASC). The photochemical damage is circumscribed to the close vicinity of the chromophore
14.4 Simultaneous Chemical and Photochemical Protein Cross-Linking of Eye Lens Proteins Incubated in the Presence of Ascorbate and Exposed UVA-Visible Light [85]
The in vitro incubation of lens proteins with ascorbic acid, which is present at high concentrations in the eye lens, results in glycation and protein cross-linking [16, 52]. Additionally, the glycated proteins are characterized by their sensitizer activity, which is very similar to that of the water-insoluble fraction from aged human lenses [48]. Colored ascorbic acid oxidation products obtained at 60 °C in oxidative conditions generate tryptophan photooxidation and glucose 6-phosphate dehydrogenase deactivation when these products are irradiated with UVA-visible light [63].
The glycating and photochemical properties of the decomposition products of ASC make them particularly useful in experiments in which eye lens proteins are incubated in the presence of this vitamin, when the solutions are in the dark and also exposed to light, using a low concentration of oxygen, simulating the physiological conditions of the eye lens. The results observed in Fig. 14.7 show that the incubations performed in the presence of UVA-visible light generate an increase in the amount of protein cross-linking compared to the samples maintained in the dark.
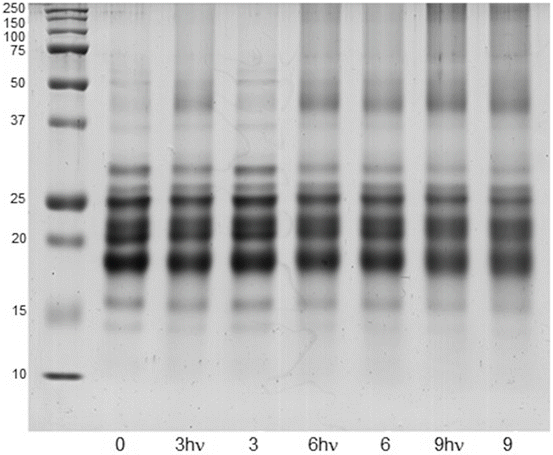
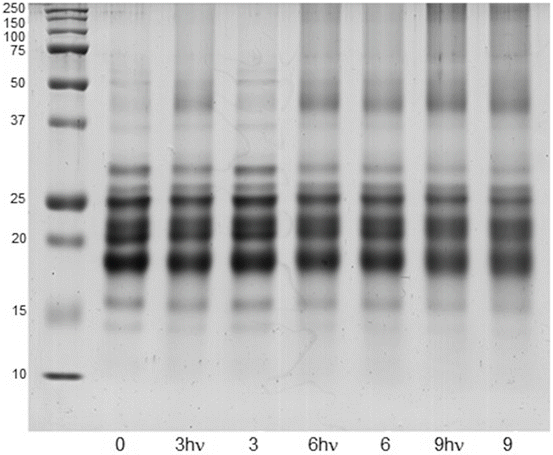
Fig. 14.7
SDS-PAGE profiles of bovine eye lens proteins (10 mg/mL) incubated during 0, 3 and 9 days at low oxygen concentration with ASC (3 mM). The samples were incubated in the absence and presence of UVA-visible light (3, 6, 9 and 3hυ, 6hυ, 9hυ, respectively). Lane 1 corresponds to a molecular weight marker. Reproduced from Photochem. Photobiol Sci. 2010, 9: 1351-1358 by permission of The Royal Society of Chemistry (RSC) for the European Society for Photobiology, the European Photochemistry Association, and the RSC
Using densitometry, it was possible to assess the increased cross-linking in the samples exposed to UVA-visible light during the incubation. The cross-linking was estimated by considering all the fractions above 37 kDa. The following quotients were obtained: (cross-linking)3Dhυ /(cross-linking)3D = 1.04; (cross-linking)6Dhυ /(cross-linking)6D = 1.16 and (cross-linking)9Dhυ /(cross-linking)9D = 1.35. When the protein samples were incubated in the dark or exposed to light in the absence of ASA, no significant modification of the electrophoretic patterns was observed. The fact that both irradiated and nonirradiated samples were characterized by the same decrease in amino group content and a similar rate of ASA consumption indicates that the additional cross-linking cannot be associated with an extended process of ascorbylation accompanied by protein aggregation. The nonoccurrence at 9 days of additional protein cross-linking when the samples were incubated under exposure to UVA-visible light in the absence of ASA indicates that colored AGEs bound to protein, which could be present in the high molecular weight aggregates, do not have photosensitizing capacity to induce these types of photo-processes (Fig. 14.7, lane 2). This result is in agreement with previously reported results [73]. Notwithstanding the fact that AGEs are efficient sensitizers of ASA photoconversion [29, 63], in this work, an increase in the decomposition of ASA was not observed as a consequence of the light because the rate of AGE formation was significantly slower than that of the ASA decomposition rate, which mainly occurs within the first 24 h of incubation. The photosensitizing capacity of other chromophores in the reaction medium, unrelated to AGEs and arising from the decomposition of ASA in secondary reactions, can explain the initially small but continuously increasing protein cross-linking over time, when the incubations were performed under UVA-visible light. In concordance with this assumption, protein cross-linking and oxidation were observed when colored solutions of ASA, obtained after 3, 6, and 9 days incubation of at 37 °C, were used as photosensitizers [85].
Scheme 14.2 summarizes the main chemical and photochemical processes that occur when bovine eye lens proteins are incubated in the presence of ASC at low oxygen concentration and maintained in the dark or exposed to UVA-visible light.
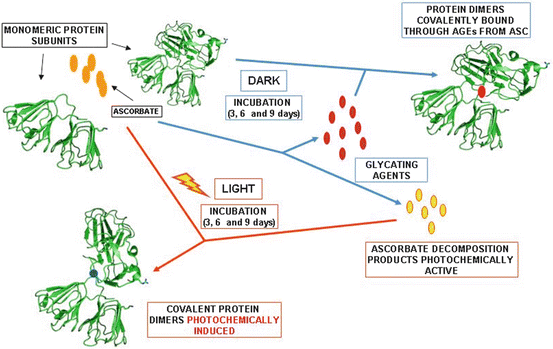
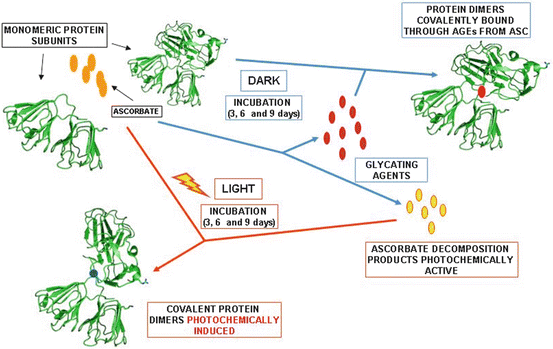
Scheme 14.2
Blue arrows show the chemical processes that occur during the incubation of bovine eye lens proteins with ASC in the dark. When the same experiment was repeated by exposing the incubation mixture to UVA-visible light, the occurrence of photochemical processes was observed (red arrows) in addition to the reactions that occurred in the absence of light (blue arrows). The experiments were performed at 37 °C, in phosphate buffer (pH 7.4), under low oxygen pressure (5 % O2)
14.5 Age-Dependent Protein Modifications and Declining Proteasome Activity in the Human Lens
The ocular lens is made up of approximately 60 % water and 38 % proteins [22, 86], which constitute almost 35 % of the wet weight of the organ and 100 % of the total dry weight. These proteins are called lens crystallins. The lens is characterized by a low molecular oxygen concentration because it lacks vascularization, and it is also characterized by a reduced cellular exchange [56, 86]. This type of environment leads to the accumulation of the modifications suffered by its structural components throughout the lifetime, which eventually leads to the aging of the organ and, in certain cases, the formation of cataracts [22].
In the aged lenses, higher molecular weight proteins tend to accumulate. The amount of these products increases as the individual gets older [87, 88].
Once synthesized, all cellular proteins of an organism have a certain lifetime and, therefore, are continuously degraded to their constituent amino acids. The lack of protein turnover in the central region of the lens causes an increase in the concentration of oxidation-modified crystallins because the greater lifetime of proteins in this zone increases the possibility that they undergo chemical modifications and oxidative stress [88–90]. The external part of the lens is protected by a large number of antioxidant enzymes, but these mechanisms are reduced in the core of the lens [91].
The damaged proteins must be repaired or degraded because the accumulation of such material is associated with malfunctioning of cells, tissues, and organs. In addition, oxidative stress, to which the eye lens is permanently exposed, is one of the causes for the aging of cellular components; in addition, several diseases [92], including cataract [93] formation [94], have been related to oxidative stress [95]. The identification and degradation of these proteins are fundamental mechanisms of cellular defense, and the proteasome is the main proteolytic complex that performs this cleaning process [96]. Proteasomes are enzymatic complexes of high molecular weight that are widely distributed in the cytosol as well as in the nucleus of the cell [97]. The proteins that are prone to be degraded by the proteasome must present one or more of the following characteristics [98]: (1) are oxidized and, consequently, are toxic for the cell; (2) are incorrectly assembled and, therefore, incapable of fulfilling their functions properly, and (3) have a short lifetime, which applies to the great majority of intracellular proteins [99]. This latter fact implies that the proteasome is involved in the regulation of the cellular cycle in plants and animals because it directly modulates the activities of other proteins within the cell [100]. Therefore, the proteasome is involved in functions that are absolutely necessary for cellular growth and viability. In mammalian cells, the 20S proteasome is constituted by four stacked heptameric rings that form a hollow cylinder. Each ring consists of seven different subunits that belong to two families, α and β, that are located in the outer and inner part of the cylinder, respectively [97]. Two activators, named 19S and 11S, can bind the 20S proteasome on each side of the cylinder and give rise to different complexes [96]. The 26S proteasome, which consists of the 19S and 20S particles, degrade proteins in a ubiquitin-dependent and ATP-dependent manner [101]. Most of the substrates that are degraded by proteasomes, especially proteins with short half-lives, must be tagged for their degradation by the addition of a polyubiquitin chain [102]. Ubiquitin is a 76-residue peptide that is present in eukaryotic cells [103]. Ubiquitin binds covalently to proteins through a highly regulated energy-dependent process [104]. The number of ubiquitin units that bind to the protein as a chain is not constant, and it is thought that the length of the polyubiquitin chain helps to fix the proteins to the proteasome entrance for the time necessary to denature it completely and, thus, prepare it for complete degradation.
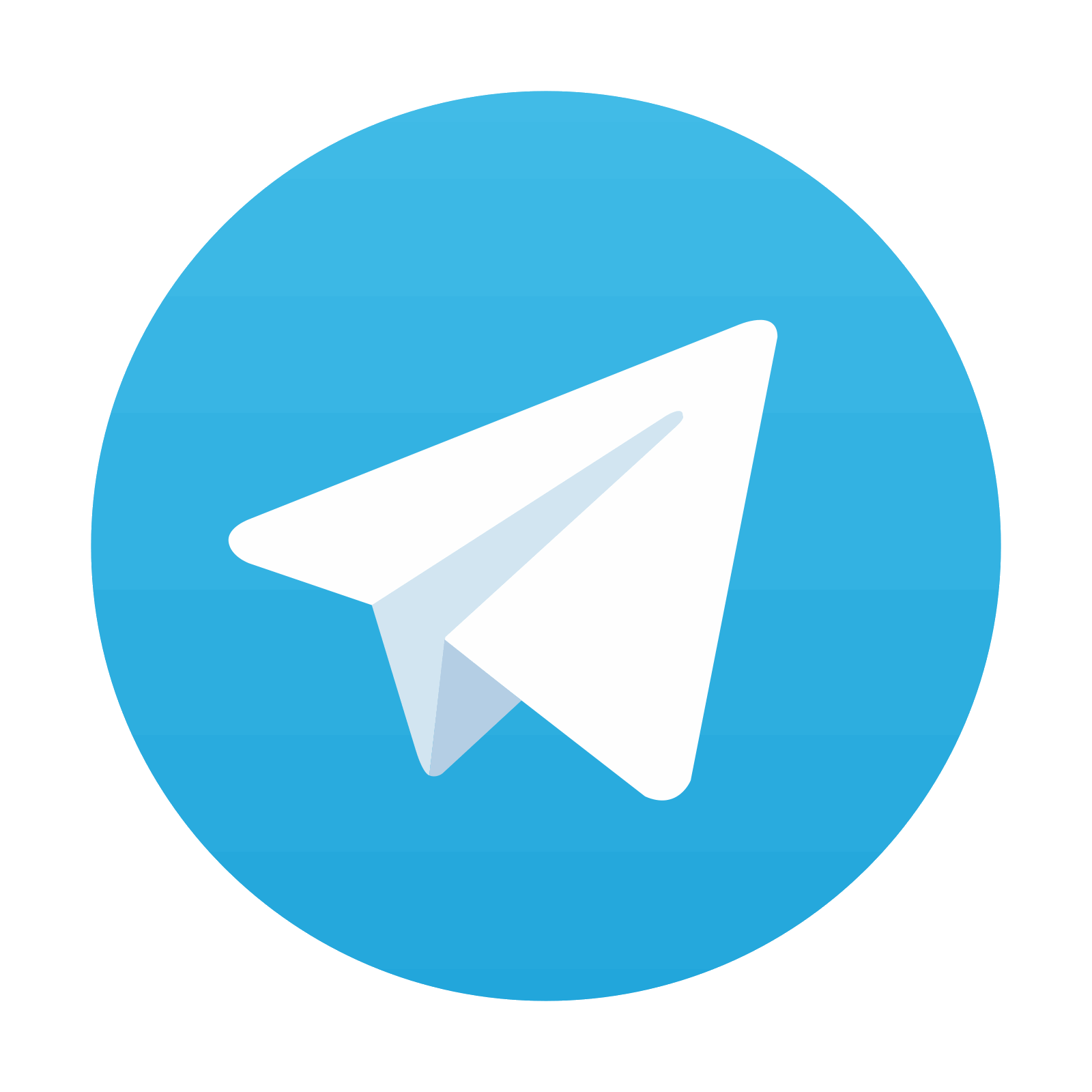
Stay updated, free articles. Join our Telegram channel
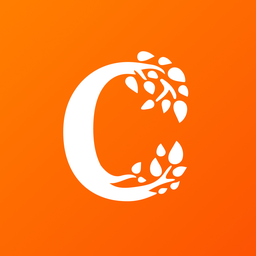
Full access? Get Clinical Tree
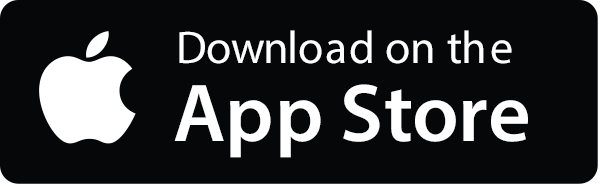
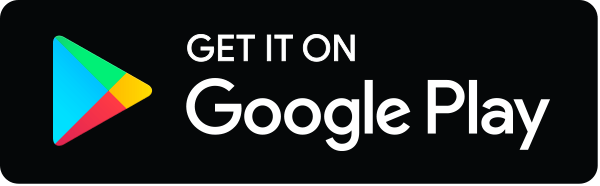