Pharmacology of Local Anesthetics and their Applications in Cataract Surgery
Adeela Malik
PHARMACOLOGY OF LOCAL ANESTHETICS
Local anesthetics are used extensively for ophthalmic,1 cutaneous,2,3 and dental4 anesthesia techniques. Modern synthetic anesthetic agents have developed5 mainly based on the evolving knowledge of the structure of cocaine containing an essential ester bond. Esters unfortunately during esterification lead to toxic para-aminobenzoic acid (PABA), and this has led to alternative amide bond type anesthetic agents, which are relatively less toxic.
The physiochemical variables such as the speed of onset and the potency of local anesthetic agents are determined primarily by their dissociation constant pka and the partition coefficient pK, respectively, in relation with the pH value of the tissue/extracellular fluid. The duration of action of the agent is determined mainly by its protein-binding capability, where the bound proteins act as a drug reservoir.
Stereochemistry is attaining greater attention in drug preparations.6,7,8 Local anesthetics contain asymmetric carbon atoms; consequently, chirality (defined as the geometric property of not being superimposable with its mirror image) is inherently involved. Most local anesthetics are produced as racemic preparations, a mixture of the two enantiomers (isomers) of the chiral molecule. However, recent studies in animals suggest that single isomers of levobupivacaine and ropivacaine provide improved safety and pharmacokinetics compared to the racemic preparations, but the definite benefits of these single isomers in humans are yet to be confirmed.
Although the developments in the chemistry and pharmacology of the local anesthetic agents and the improvements in operative conditions have led to reductions in the incidence of adverse events, an inappropriate use of these agents can lead to adverse consequences. To minimize risks, it is vital for the clinician to have a thorough understanding of the physiology of neuronal function, the chemistry and pharmacology of various local anesthetic agents, and the potential of these agents to cause both local and systemic toxicity.
Background/History
Historically,9 ocular procedures have had an enormous influence on the discovery of anesthetic modalities. Cocaine is the oldest naturally occurring local anesthetic, having been in medical use5,10.11 since 1862. It is an alkaloid derived from the leaves of the Erythroxylon coca bush. In 1884, Carl Koller and Sigmund Freud used it as an anesthetic agent during ophthalmic procedures. However, its inherent toxicity limited its universal uptake by the ophthalmic community. It had a low therapeutic ratio, which could result in convulsions and even death when injected as a regional anesthesia. Procaine, a synthetic alternative to cocaine, was developed in 1905. It is an ester derivative of PABA and during metabolism, PABA (a strong allergen) is released back as a metabolic product, and this limits the use of procaine and other ester-type anesthetic agents. Tetracaine (Amethocaine), another ester type anesthetic, was introduced in 1930. It is more potent than procaine and also causes allergic reactions.
In 1943, lidocaine, an amide derivative of diethylaminoacetic acid, was introduced. Generally amide derivatives are less allergenic, and multiple amide-type anesthetics have been introduced into clinical use with slight chemical alterations to the compounds that have yielded beneficial characteristics, including increased duration and potency. Single-isomer preparations such as levobupivacaine and ropivacaine (both amide types) have shown great promise in the lab and in animal trials but have yet to demonstrate clinical benefits, especially in toxicity in humans.
Chemistry/Structure
Structurally, local anesthetics consist of three molecular components12,13,14,15,16,17: a lipophilic (aromatic ring), an intermediate aliphatic chain, and hydrophilic amine derivatives (Fig. 35-1). The intermediate hydrocarbon chain is joined to the amine moiety by ester (COO) or amide (CONH), and is the general basis for classification of local anesthetics. Each constituent molecular component may be modified to achieve a specific function.
![]() Figure 35-1. General structure (three molecular components: aromatic ring, intermediate chain, and amine group) of local anesthetics. |
Generally, electron-donating groups on the aromatic ring increase and the electron-accepting groups reduce the duration of activity of the agent. Adding a second substituent to the aromatic ring increases the activity of the agent.
Increasing the length of the intermediate chain (at the alpha carbon) increases both activity and toxicity. Unlike in amides, branching in esters increases the duration of action, and the carbonyl must be in conjugation with the aromatic ring.
Modifications to the terminal amine, leading to primary, secondary, tertiary, and quaternary forms, are possible. The tertiary form (-NH2) is lipid soluble, whereas a quaternary form (-NH3+) is positively charged and renders the molecule water soluble.
Figure 35-2 illustrates the structural details of procaine (ester), cocaine (ester), and lidocaine (amide) anesthetic agents. Table 35-1 lists some of the common ester- and amide-type anesthetic agents.
![]() Figure 35-2. Molecular diagram of typical ester (procaine and cocaine) and amide (lidocaine) local anesthetic agents. |
Table 35-1. Physiochemical and Chemical Properties of Various Local Anesthetic Agents | ||||||||||||||||||||||||
---|---|---|---|---|---|---|---|---|---|---|---|---|---|---|---|---|---|---|---|---|---|---|---|---|
|
Ester-type anesthetics are metabolized, and the degradation process gives rise to PABA metabolite, which is highly antigenic and a source of allergic reactions. Amides, compared to esters, are normally much more stable in plasma.
Other molecular structural details of the anesthetic agent also affect its properties. Generally both larger alkyl substitutions on the nitrogen atom of the hydrophilic group and lengthening of the intermediate chain increase the activity of the anesthetic agent, and the latter also leads to increased toxicity.
Most anesthetic drugs are chiral6,7,8 (a geometric property of not being superimposable with its mirror image). In organic molecules this arises mainly due to asymmetric arrangement of carbon atoms. The two enantiomers of chiral drugs are best identified on the basis of their absolute configuration (determined by precise rules based on atomic number and mass) or their optical rotation of the polarized incident light. R/S convention for absolute configuration and +/- convention for optical rotation is often used. (R indicates rectus, meaning to the right, and + denotes clockwise direction; S indicates sinister, meaning to the left, and – indicates counterclockwise direction.)
The enantiomers of a chiral drug have identical physical and chemical properties in an achiral environment, but in living systems (which are chiral) each of the enantiomers of chiral drug can behave very differently in vivo: One enantiomer may be responsible for the therapeutic effects, and the other may be inactive or contribute to undesirable effects.
Bupivacaine, etidocaine, mepivacaine, prilocaine, and ropivacaine are all chiral due to asymmetric carbon atoms, and most of these are produced as racemic, i.e., containing both isomer mixtures (R and S conformal configurations). Studies on the anesthetic activity and toxicity of the individual enantiomers of bupivacaine and mepivacaine indicate that the single isomers (S enantiomers) are long acting and less toxic than the R enantiomers.15,16
Mechanism of Action
Local anesthetics reversibly block the conduction of action potential in neurons by interacting with the pores of the voltage-gated sodium channels.17,18,19,20 This site is intracellular, so the injected local anesthetic must first defuse through the lipophilic cell membrane.
Most synthetic anesthetics are a weak base,19,20 and during manufacture precipitate as powdered solids that are unstable in air and poorly soluble in water. Therefore, the solids are usually combined with an acid to produce an injectable and stable acidic (hydrochloride) salt solution (pH 3–6). Thus all anesthetic solutions are acidic (BH) prior to injection, and after injection the solution quickly takes on the pH value of the surrounding tissue and is rapidly reduced to cationic form and exists in equilibrium as a quaternary salt (BH+) and tertiary lipid-soluble base B:

The molecular structure thus shifts between uncharged tertiary base molecule B (lipid-soluble) and positively charged cation (BH+). The equilibrium is determined by the exact pH and the dissociation constant pKa of the solution in accordance with the Henderson-Hasselbalch equation:

If the surrounding medium becomes more acidic (i.e., lower pH), then the positively charged ions (BH+) combine with the uncharged anesthetic molecule (B), shifting the equilibrium to the left and thereby producing a higher proportion of charged cationic structures (BH+). If the pH rises (that is, the solution becomes more alkaline and there are fewer positively charged hydrogen ions), the charged radicals are released into the solution and the equilibrium shifts to the right, producing more uncharged base (B). Only the uncharged form (B) is able to diffuse through the lipid membrane of the cell. Inside the cell, positively charged base molecules (BH+) tend to combine with the sodium channel proteins and are removed from the dynamic balance, creating a demand whereby more and more neutral base (B) molecules from outside the cell are drawn in. Thus inside the cell the equilibrium shifts to the left to recreate the original concentration of cations and neutral based molecules.
As more and more of the uncharged base (B) diffuses through the membrane, the concentration of the uncharged base outside the membrane decreases and the equilibrium shifts to create more uncharged base (B) from newly higher concentrations of positive cations BH+. This process continues until eventually nearly all the base diffuses from the outside of the cell membrane to the inside. The operating values pH and pKa of the aesthetic agent are important and determine the onset speed and duration of the anesthetic. In comparison with the physiologic pH (7.4), the disassociation constant (pKa) of all local anesthetics exceeds 7.4. The pH of normal body tissue is 7.4, and in the presence of an active infection, the tissue pH can be considerably lower (about 5–6), but for an inflamed tissue the pH generally remains normal at 7.4.
Physiology of Nerve Conduction
Neuronal factors such as the axon diameter and myelinization determine the relative interaction of the neurons with the anesthetic agent.21,22,23 For larger neurons, the duration of the anesthetic action is reduced. The penetration of anesthetic is decreased with increasing amount of myelin. Based on the size of their diameters, the nerves are categorized into three types: A, B, and C. The largest (type A) conducts pressure and motor sensations. The medium size (type B) is myelinated, and the smallest (type C) is unmyelinated. Type C neurons transmit pain and temperature sensations. Anesthetics normally block type C nerves more easily; thus patients with type C block for pain still feel pressure and have mobility as type A fibers are not blocked.
Pain sensations (and for that matter any sensory signal) propagate along the nerves in the form of a traveling electrical wave called action potential. Normally, an action potential elicited at any one point on an excitable membrane excites adjacent portions of the membrane, resulting in the propagation of the action potential.
The action potential wave arises21,22 due to the sequential variations in the membrane conductance related to the conformational changes in the channel and is designated in terms of resting (polarized), activation (depolarization), inactivation (repolarization), and deactivation states of the membrane (Fig. 35-3), mediated by the action of the various membrane ion gates (Fig. 35-4).
In the resting state, chemical and electrical gradients exist across the neuronal membrane, giving rise to resting potential of (-90 mV) at the inside of the membrane with respect to the outside. These potential gradients are established through the function of the various passive sodium and potassium ion leak channels including Na+/K+ ATPase channels. During polarization, i.e., the buildup to the resting potential -90 mV, the inward leakage of Na+ ions and the outward leakage of the K+ ions along the leakage channels is in the ratio of 1:100 and gives rise to a potential of -86 mV. The 2-pore domain potassium (K2P) channels are responsible for the K+ ion leakage.20,24,25 This is further depressed to -90 mV through the action of the active Na+/K+ ATPase pump where Na+ and K+ ions are pumped in and out of the membrane, respectively, in the ratio of 3:2.
The activation (or depolarization) of the membrane occurs due to a sudden influx of Na+ ions into the interior of the membrane and is regulated primarily by the voltage-gated sodium channels sensitive to membrane potential. The outflow of K+ ions, regulated by voltage-gated K+ channels, is also involved. The voltage-gated sodium channels are equipped with activation and inactivation gates (Fig. 35-4), whereas the potassium channel has only one activation gate.
Inactivation (repolarization) occurs as a result of timely closure of the voltage-gated sodium channels, and also the opening of slow-acting voltage-gated potassium channels. During this phase there is no inward movement of sodium ions through the voltage-gated channel, and the resting potential is restored by the outward leakage of the K+ ions via the potassium voltage-gated channel.
During deactivation, the gates of the voltage-gated channels revert back to the original resting state.
Local anesthetics inhibit the depolarization of the membrane potential by disrupting both sodium and potassium ion currents, and consequently the action potential is not propagated as the threshold level is never attained.
In detail, each voltage-gated sodium channel consists of an intercellular pore that has a binding affinity for the local anesthetic.18,19,20 It is composed (Fig. 35-5) of one α-subunit or one or two β-subunits. The α-subunit in turn is composed of four domains (D1–D4), and each of these domains comprises six transmembrane helical segments (S1–S6). Anesthetic agents interact with the D4 domain and S6 transmembrane of the α-subunit of the pore and thereby inhibit propagation of the action potential.
In addition to the action of the ionized species of local anesthetics on the intracellular part of the transmembrane sodium channel, the neutral species of the anesthetic agent also affects the transmembrane, leading to an expansion of the lipid layer.
Speed of Onset of Anesthetic Agents
The speed of onset of an anesthetic agent is determined largely12,15 by its dissociation constant (pKa) and the pH value of the extracellular fluid, and as dictated by the Henderson-Hasselbalch rate equation (see previous section, Mechanism of Action).
The pKa value of most local anesthetic agents is in excess of the pH (7.4) of the extracellular fluid; thus for these agents the ionized species dominate soon after injection, and these are unable to penetrate the cell membrane. However, for agents with lower pKa and close to pH 7.4 (of the extracellular fluid), there exist an appreciable number of unionized species, and thus these agents diffuse more rapidly into the nerve cell and thereon to the pore of the sodium channel to block depolarization of the neurons. According to the Henderson-Hasselbalch rate equation, increasing pH increases the formation of more neutral species, but here the chemical stability is reduced. For an infected tissue where pH is reduced to 5 or 6, the anesthetic is obviously less effective.
Potency of Anesthetic Agents
The potency of an anesthetic agent is determined by the lipid solubility represented by the partition coefficient (pK), which is a measure of the relative distribution of the anesthetic agent between an aqueous phase and nonionized solvent (such as octanol, heptane, hexane or in the present case the lipid-bilayer) phase. Ionized species are generally aqueous soluble and unionized species tend to dissolve within a lipid–layer. For a buffered system, the relative anesthetic concentration in ionized to unionized species may be determined using the rate equation:

With an increase in the partition coefficient (pK), the ratio of the second term on the LHS of the rate equation must decrease to maintain the balance (i.e., the denominator must increase). Thus, hydrophobic drugs become more lipid soluble, resulting in an increased concentration of the anesthetic drug within the neuron membrane. As shown in Table 35-1, anesthetic agents with high pK values are therefore relatively more potent.
Duration of Action of Anesthetic Agents
Local anesthetic agents bind12,15 to both tissue and plasma proteins (albumin, α1-acid glycoprotein). The binding to albumin is considered as a high-volume low-affinity binding process, whereas α1-acid glycoprotein is a low-volume and high-affinity process. Proteins thus act as a reservoir for the anesthetic drug. Although it is the free drug that is active and relevant, studies show that the amount of protein binding correlates well with the duration of action of local anesthetic agents. Other factors such as potency, dose, and presence of vasoactive substances and vascularity of the tissue are also relevant. The addition of epinephrine to some local anesthetic solutions prolongs the duration of action due to resulting vasoconstriction and decrease in systemic absorption.
Metabolism of Anesthetic Agents
Ester-type anesthetics are hydrolysed12,13,15 rapidly by tissue and plasma cholinesterases, giving rise to para-aminobenzoic acid (PABA), which is allergenic. An exception, however, is cocaine, which is metabolized more slowly in the liver.
Amides are more stable in plasma than esters. They are metabolized by microsomal enzymes located in the liver, although a small percentage is also cleared by renal mechanisms. The specific microsomal enzyme responsible for the elimination of lidocaine (amide) is cytochrome P-4530A4. Hence amide-type anesthetics should be used with care in patients with severe liver disease or in patients taking medication that interfere with the metabolism of the anesthetic. These patients should be monitored carefully for signs of toxicity.
The rate of metabolism depends heavily on liver blood flow15 and differs between agents: Prilocaine and etidocaine are the most rapid, lidocaine and mepivacaine are intermediate, and bupivacaine and ropivacaine are the slowest. The clearance of prilocaine exceeds what the liver could do alone, and this suggests that extrahepatic mechanisms are also involved. Beta-blockers may decrease blood flow to the liver; therefore, they may also decrease the metabolism of amide-type anesthetic agents and result in increased anesthetic levels in the serum.
Toxicity
The toxicity of local anesthetic agents mainly affects the central nervous system (CNS) and cardiovascular system.
Direct injection27,28,29 of an anesthetic agent into a muscle or nerve can give rise to local toxicity whereas systemic toxicity occurs due to an inadvertent intravascular injection or absorption of excessive amount of anesthetic agent.
Local anesthetics can readily cross the blood-brain barrier and produce CNS excitation and depression. CNS toxicity, following rapid intravenous injection of local anesthetic agent, is related to the intrinsic anesthetic potency of the agent, which in turn is a function of the partition function. As shown in Table 35-1, procaine is the least potent and also least toxic. Lidocaine, mepivacaine, and prilocaine are intermediate both in potency and toxicity. Bupivacaine and etidocaine are most potent and consequently also most toxic.
Neurotoxicity
Direct injection15,28 of anesthetic into a nerve, or where the nerves are bathed in highly concentrated solution of anesthetic over a prolonged period of time, gives rise to neurotoxicity. It may also occur as a side effect with concentration of lidocaine normally used for the treatment of ventricular tachydysrhythmias. In vitro studies15 for the estimation of the strength of lidocaine for the onset of neurotoxicity resulted in 2% for squid axon models compared to 5% in patients (using spinal microcatheters). The underlying mechanism here is the bathing of the nerves in concentrated lidocaine agent over a prolonged period of time. However, as in ophthalmic regional anesthesia, neither highly concentrated anesthetic nor a prolonged contact of the agent with the nerves is expected to occur. Thus any neurologic effects occurring during this modality may not be attributed solely to the anesthetic agent. Coexisting vascular pathology is also involved, possibly complicated by the use of highly concentrated local anesthetic containing vasoconstrictor additives that develop high orbital pressure.
Myotoxicity
Injection of an anesthetic agent directly into a muscle15 causes necrosis, where subsequent fibrosis and contracture of the muscle lead to diplopia. Here often inferior oblique, inferior rectus, and medial rectus muscles are involved.
Myotoxicity has also been reported in sub-Tenon procedures, where it is believed that the agent penetrates into the muscle via small fenestrations in Tenon fascia. The risk of local anesthetic–induced mild toxicity may be reduced by the addition hyaluronidase, which helps to disperse the anesthetic agent away from the muscle.
Corneal Epithelium Toxicity
High or prolonged doses of local anesthetic agents are toxic to corneal epithelium;30 whereby wound healing is prolonged and corneal erosion could also occur. Repeated drops of agent can cause clouding of the cornea, rendering surgery more difficult. Tetracaine is the most irritating of the available eye drops.
Systemic Toxicity
Systemic effects occur when the concentration of the local anesthetic in the blood increases to toxic levels.
Although in ocular regional anesthesia, systemic reactions are uncommon,15 these may, however, occur when the agent is injected through the dural cuff into cerebrospinal fluid around the optic nerve. Here brainstem anesthesia could occur along with loss of consciousness and respiratory and cardiovascular depression. For topical anesthetics, the maximum permissible dose should be adhered to and great caution exercised when using topical anesthetics on mucosal surfaces, which can lead to increased absorption.
Systemic toxicity due to anesthetic agents manifest as adverse reactions29 in both the CNS and the cardiovascular system. The CNS is affected in a predictable and dose-related manner, and the effects are aggravated with an increase in the serum level.
CNS excitation is related to the inhibition of the cerebral cortical neurons. Initially, selective blockade of inhibitory cortical neurons or synapses permit facilitatory fibers to function unopposed, leading to excitation and convulsions. Further increase in dosage depresses both inhibitory and facilitatory pathways leading to a generalized state of CNS depression.
The sequence and symptoms of systemic toxicity vary according to the level of serum toxicity: At a lower level (e.g., lidocaine 1–5 μg/mL) the patient may complain of circumoral and tongue paresthesia, metallic taste, and dizziness; at an increased level (e.g., lidocaine 5–8 μg/mL), nystagmus, slurred speech, localized muscle twitching, or fine tremors are noticed, and the patient may even have hallucinations at these levels; at elevated levels (e.g., lidocaine: 8–12 μg/mL), focal seizure activity occurs, and this can progress to generalized tonic-clonic seizures. At extremely high toxicity levels (e.g., lidocaine 20–25 μg/mL), respiratory depression occurs and can progress to coma.
Supportive treatment consists of securing the airway and using anticonvulsants to control seizure activity.
At an elevated and increased rate of rise of plasma concentration, the neuron effects are accompanied by cardiovascular complications.31 A decrease in cardiac function can lead to hypotension, needing advanced cardiac support protocols. Impending toxicity may be signaled by bradycardia with prolonged PR interval (i.e., the time from the beginning of the onset of atrial depolarization to the beginning of the onset of ventricular depolarization) and broad QRS complex (electrocardiogram [ECG] representation of the passage of the heart’s electrical impulses through the ventricles). This may be followed by a range of dysrhythmias such as heart block, multifocal ectopics, tachycardia, and ventricular fibrillation. Thus the treatment is supportive, including antiarrhythmics such as amiodarone, phenytoin, and bretylium.
To reduce CNS toxicity, steps should be taken to reduce hypoxia and acidosis. The patient’s airway should be maintained, as an increase in blood levels of carbon dioxide leads to a decrease in protein binding of amides (lidocaine), resulting in higher levels of free lidocaine in the blood. Increased respiration and alkalosis increase the seizure threshold and decrease the uptake of the local anesthetic into the CNS. If convulsions occur, the patient’s airway should be maintained with supplemental oxygen.
Although lidocaine has a role in the treatment of ventricular tachydysrrhythmias, it is advisable to avoid it if local anesthetic cardiotoxicity has been established.
Systemic reaction can also result from injection of a much smaller dose of anesthetic agent but at a high pressure into the arterial system, leading to retrograde spread of concentrated anesthetic solution directly to the brain.
A comparative study for the dose-related toxic effect of intravenous bupivacaine, ropivacaine, and lidocaine in sheep15 resulted in the ratio of 1:2:9, respectively. The lidocaine-treated group of sheep died of respiratory depression, bradycardia, and hypotension, but without arrhythmias. The bupivacaine-treated group died of ventricular arrhythmias in the absence of hypoxia or acidosis. The ropivacaine group died from a combination of these factors or as a result of sudden onset of arrhythmias alone.
Clinical remedial measures for reducing/minimizing systemic toxicity complications include the following: careful positioning of the injection needle, aspiration before injection, adequate time between doses, use of less toxic agents, and use of additives such as hyaluronidase to reduce the amount of anesthetic required or epinephrine to counter the direct vasodilation caused by most local anesthetics.
Allergy
Allergic reactions32 to local anesthetics and especially to amide-type anesthetics are extremely rare. No cross-reactivity appears to exist between ester and amide anesthetics.
Allergic reactions can be type 1 (i.e., anaphylactic) or type 4 (i.e., delayed type hypersensitivity reactions), and these reactions are not dose related.
Type 1 reactions are caused by ester-type anesthetics, and cross-reactivity exists among ester anesthetics. The reactions are normally triggered by PABA, a metabolite of ester hydrolysis. Clinical signs of type 1 reaction include pruritus, urticaria, facial swelling, nausea, vomiting, and abdominal cramping. Treatment includes epinephrine at a concentration of 1:1,000 administered subcutaneously at a dose of 0.3 to 0.5 mL, repeated every 20 to 30 minutes to a maximum of three doses. If anaphylaxis persists, a 5-mL dose of epinephrine 1:10,000 should be administered intravenously.
Type 4 (delayed hypersensitivity reactions) accounts for 80% of allergic reactions to local anesthetics and occur as a result of topical applications of subtypes of amide and ester anesthetics. Clinical manifestations are similar to that of allergic contact dermatitis and include erythema, plaques, and pruritus. The treatment for contact dermatitis includes topical steroid preparations.
Alternative anesthetic treatments for patients with known allergy with either ester- or amide-type local anesthetics include use of isotonic sodium chloride solution and injectable antihistamines such as (intramuscular) diphenhydramine. Injection of diphenhydramine is painful but sedating.
Reactions to Drug Additives
All local anesthetics directly cause relaxation33 of vascular smooth muscle leading to vasodilatation, which in turn promotes increased bleeding at the surgical site. Vasoconstrictors such as epinephrine are often added to negate bleeding.
The effectiveness of epinephrine is maximal at 15 to 17 minutes and is evidenced by blanching of the skin.
Vasoconstriction also reduces the rate of systemic absorption of the anesthetic, therefore allowing the body more time to metabolize and hence prolong anesthesia. Thus addition of epinephrine permits injection of increased volumes of anesthetic agent.
Epinephrine
Epinephrine is contraindicated in patients with pheochromocytoma, hyperthyroidism, and severe hypertension or severe peripheral vascular occlusive disease. Relative contraindications include pregnancy and psychological instability.
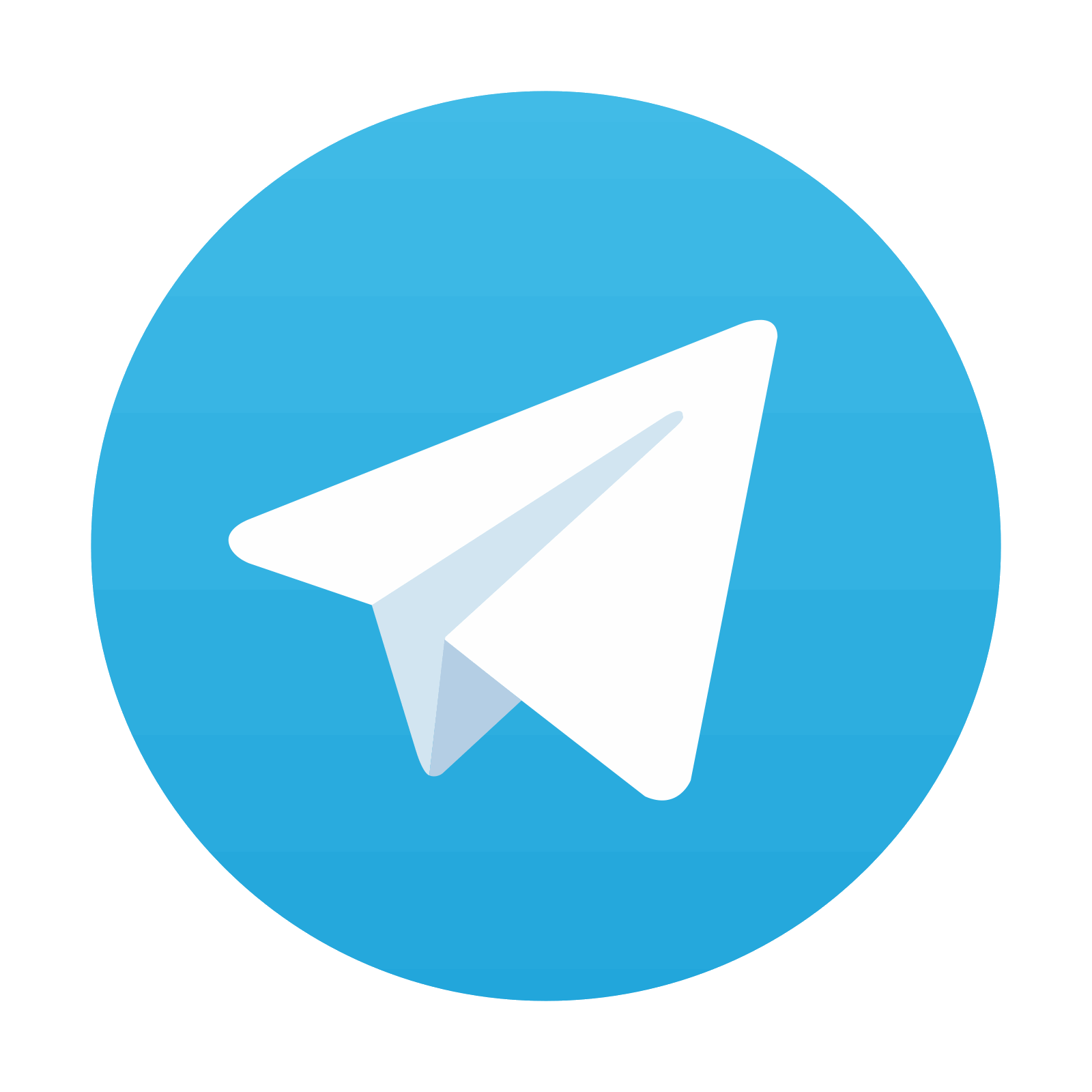
Stay updated, free articles. Join our Telegram channel
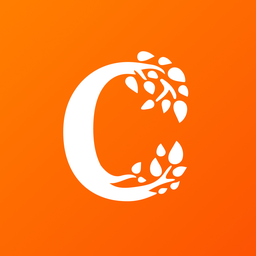
Full access? Get Clinical Tree
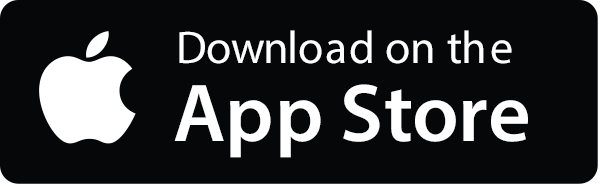
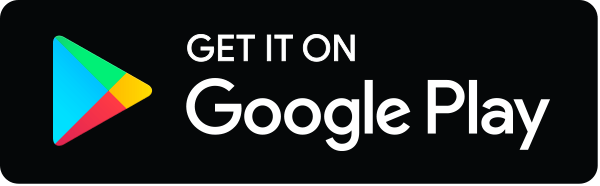
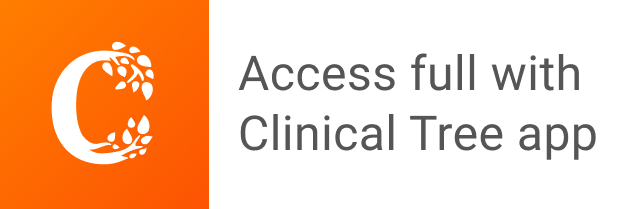