Fig. 1
Vocal cord paralysis. Upper airway obstruction due to vocal cord paralysis noted on bronchoscopy. Courtesy of Steve Sobol, ( The Children’s Hospital of Philadelphia)
Tracheobronchomalacia (TBM) is a dynamic airway narrowing disorder that is difficult to diagnose. The estimated incidence is between 1 in 1,500 and 1 in 2,500 in children, which is thought to be a significant underestimation of the true incidence. This condition has been reported in up to 15 % of infants and 30 % of young children (≤3 years old) who have undergone bronchoscopy for evaluation of respiratory distress [31–33]. In the selected population of premature infants with BPD, incidence between 16 and 50 % has been reported [34]. Identified risk factors associated with the development of secondary TBM include lower gestational age at birth, prolonged intubation, higher mean airway pressures during mechanical ventilation, infection, longstanding extrinsic airway compression (from vascular structures, cardiac and skeletal abnormalities, and tumors/cysts), and chronic inflammation [20, 35].
The incidence of small airway disease varies with BPD with an incidence of 68 % in children with history of BPD, 30 % demonstrating moderate degrees of obstruction and 10 % demonstrating severe obstruction on lung function tests.
Pathogenesis
In comparison with tracheas of infants who have not been ventilated mechanically, tracheas of those who have been ventilated are less compliant, being more difficult to expand but easier to collapse. Both tracheomegaly [36] and tracheomalacia [37] have been described in preterm infants who required mechanical ventilation in the neonatal period. Tracheomalacia may occur as a consequence of epithelial disruption with loss of an epithelial relaxant and an intensive bronchoconstrictor response to inhaled agonists [38, 39]. Disruption of the muscle–cartilage junction may reduce the ability of the trachea to resist compressive forces such as high supra-atmospheric pressures produced in infants with airflow obstruction. Pressure-induced alterations in the orientation of airway smooth muscle fibers may affect the forcefulness of smooth muscle contraction and the effectiveness with which muscle contraction stiffens the airway and helps it resist deformation. It is also possible that pressure-induced alterations in the alignment of cartilage components (i.e., proteoglycan/collagen configuration) results in cartilage providing less structural support for the trachea in newborns who have been mechanically ventilated.
The trachea and large airways are very compliant during development, with compliance of the airway determined largely by the tracheal smooth muscle contained in the posterior wall [40, 41]. During mechanical ventilation, alterations in tracheal smooth muscle mechanics in preterm [41], newborn lamb [42], and adult dog [43], often parallel changes in tracheal compliance seen with lung development. The role of tracheal cartilage in determining tracheal compliance is less well defined. The primary contribution of tracheal cartilage in determining tracheal compliance and dimensions appears to involve its role in limiting evagination of the posterior wall when transmural pressure is positive and invagination when transmural pressure is negative. During spontaneous respiration and lung inflation, a decrease in intrathoracic pressure increases transmural pressure in the intrathoracic trachea, causing the posterior tracheal wall to evaginate and the cartilaginous tips to be pulled further apart. The increasingly inward forces generated by the cartilaginous tips resist this expanding force, thereby limiting the increase in intraluminal area in the intrathoracic trachea. During exertional expiration, when intrathoracic pressures are relatively positive and transmural pressures in the intrathoracic trachea may be negative, posterior wall invagination and tracheal compression is limited by an outward, and therefore stabilizing, force exerted by the ring [14]. In the immature animal, a reduced ability of the cartilaginous ring to exert these forces may contribute to age-related differences in tracheal compressibility and inflatability. The net effect of increased airway compliance is a change in the pressure-flow relationship, which contributes to the high airway resistance and limitation to airflow [41]. Along with high airway resistance and limitation to airflow, increased airway compliance allows for acute and chronic airway deformation with exposure to positive pressure. With exposure to positive pressure tracheal cross-sectional area, circumference, and mean diameter were found to be significantly greater. The greater circumference was mainly due to the greater length of the trachealis muscle whereas the cartilage length remained essentially unchanged [44]. Other studies have subsequently confirmed these findings with demonstrating with 4 h of ventilation a time-dependent decrease in posterior wall thickness, indicating a thinning of the trachealis muscle and no detectable change in anterior wall thickness, indicating a relative stability of the cartilage component of the tracheal boundary [45]. The radius of the trachea from preterm infants is approximately half that of the adult. The LaPlace relationship, therefore, suggests that the preterm trachealis needs to generate a tension half that of the adult to withstand an equal deforming pressure (Fig. 2).
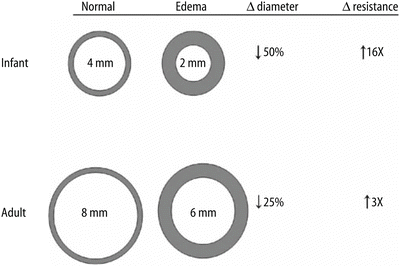
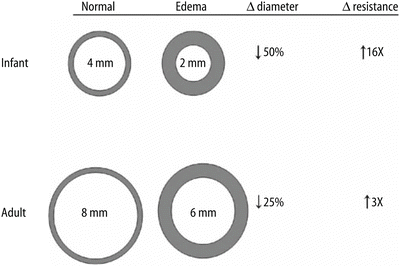
Fig. 2
Differences in luminal size and airway resistance with 1 mm circumferential edema: Changes in airway resistance with intraluminal swelling are more pronounced in infants compared with adults. (Adapted from Wheeler et al. [117]. In Wheeler et al., Resuscitation and Stabilization of the Critically Ill Child)
When immature airways are exposed to distending pressures associated with positive pressure ventilation (PPV), they become deformed, although adult airways do not [46]. This pressure-induced injury to the immature airway may arise in part because of the inability of the preterm trachealis muscle to generate enough tension to withstand that level of barotrauma. The clinical consequences of decreased airway compliance include increased dead space, flow limitation, elevated airway resistance and work of breathing, and higher risk of gas trapping. Thus, the limited ability of immature airway smooth muscle to generate tension may help explain why preterm neonates who require PPV are at risk for developing structural injury to the central airways.
Contrasting the trachea, where compliance progressively decreases from the early fetus to the adult, studies have shown no difference between 1-month-old and adult bronchi with respect to deflation-specific compliance [47]. However as a fetus and in the first month of life, the bronchi are twice as distensible as they are at a month of life. Airway morphology was investigated in an attempt to identify structural changes that might produce the observed maturation of specific compliance. Bronchi from 1-week-old pigs had a significantly lower total wall area and cartilage area than the fetus and 4 week olds. However, the percentage of cartilage in the wall of fetal, 1- and 4-week-old pigs was the same. The mechanism for age-related changes in compliance could involve either a change in composition of the wall (e.g., more cartilage) or a change in the mechanical properties of some wall component (e.g., stiffer cartilage), but is not related to changes in airway smooth muscle tone. Fetal pig bronchi had the same proportion of cartilage as 4 week olds but were significantly less compliant, implying that the maturational change in compliance was not explained by changes in the amount of bronchial cartilage. Cartilage and smooth muscle stiffness are important determinants of the maturational change in tracheal compliance [48, 49], but in bronchi the structures determining specific compliance are unknown. Both immature and adult bronchi showed a change in the internal area (Ai) with inflation, however, no statistically significant change in the outer area (Ao) or total wall area (WAtot) of adult bronchi was detected with inflation. As with the adult, no difference in Ao was detected between inflated and uninflated bronchi from 1-week-old piglets; however, inflation to 20 cmH2O did produce significant reductions in the wall thickness and total wall area (WAtot) of 1-week-old bronchi; therefore, at this pressure, part of the increase in lumen volume occurs by compression of the airway wall. Some expansion of Ao and movement of the cartilage plates may occur, but at least in bronchi from immature pigs, airway wall compression is a factor in determining lumen volume and specific compliance. Airway mucosa and smooth muscle are incompressible structures [50]; therefore, the region of wall compression may lie in the submucosa between the muscle and cartilage layers. This region can expand during muscle constriction in vitro, suggesting that it may also be compressible [51]. In vitro, fluid appears able to enter the wall of isolated bronchi across their adventitial surface, whether this can occur in the lung in vivo, is questionable.
Ventilatory strategies such as continuous positive airway pressure (CPAP) and mechanical ventilation have been implicated in the pathogenesis of diverse airway disorders [44, 46, 52]. While necessary to sustain life at the extremes of prematurity, positive airway pressure imposes physical forces on the airway that are not normally present during development. Relatively stiff lungs are mechanically ventilated through relatively compliant airways resulting in marked airway deformation. Pressure-induced stretch and high shear forces alter the normal structure and function of the airway. Anatomically positive airway pressure increases the dimensions of the trachea in the anterior–posterior diameter and the tracheal volume resulting in tracheomegaly and increasing dead space [36, 45, 53, 54]. The greatest deformation in trachea dimension is associated with the posterior region where the cartilaginous tips are spread apart. The hinged area is a high stress point where tension (outer region of trachea) and compression (inner region of trachea) simultaneously applied during exposure to positive pressure. Ultrasound technology allows precise measurement of tracheal dimensions in vivo; allowing for a comparison between changes in mechanical properties of the airway and structural changes of the airway [45, 53]. Cartilage plays a major role in the structure of the airway and while the net effect is an increase in the dimensions of the trachea, controversy exists as to whether mechanical stress during ventilation, is damaging to the chondrocytes or if this stress has an impact on airway chondrocytes and extracellular matrix (ECM) expression. Recently published data proposes changes in airway chondrocytes and ECM secondary to the effects of positive pressure on the airway as responsible for acquired tracheomegaly [55] and increased dead space. Histologically, applying positive pressure to the airway results in a thinner and stretched, pseudostratified, ciliated columnar epithelium wall as well as radial expansion of the entire tracheal cartilage and muscle [55]. Fourier transform infrared imaging spectroscopy is a novel imaging methodology that has been utilized to examine various types of natural tissues including bone, cartilage, and tissue-engineered, cartilage-like tissue, and biomaterials. The specific molecular components in cartilage contribute to its infrared spectrum, and these can be detected using FT-IRIS methodology. An FT-IRIS spectrometer, when coupled with a light microscope, enables one to identify intensity and distribution of tissue compositions. FT-IRIS technology allows for assessments of functional alterations in trachea dimensions and mechanical properties. Utilizing this technology, tracheal cartilage can be assessed with respect to collagen, PG, and integrity of newly synthesized collagen [55]. FT-IRIS analysis demonstrated differences in intensity and distribution of ECM in the tracheal cartilage with an increase in the collagen and proteoglycan content and increased signal for collagen integrity (new tissue formation). These findings demonstrate that exposure to positive pressure changes the ECM composition and the level of constitutive ECM components in the neonatal tracheal cartilage, suggesting both airway damage and a concomitant repair.
Another component of pulmonary mechanics is described in terms of compliance of an elastic organ as volume (strain) per unit of pressure (stress), where compliance is lower when the elasticity is greater. Moduli of elasticity are typically used to describe material not intended to be compliant, in this case a large conducting airway. Bulk and elastic moduli represent the amount of force (stress) to achieve a degree of deformation (strain), and thus if something is less elastic, it requires more stress per unit of distortion and has a greater moduli of elasticity. The trachea normally has some degree of elasticity, however over time, with exposure to positive pressure more force is needed to distend the airway. In this regard, the airways are loosing elasticity in response to positive pressure whereby the enlarging internal diameter is associated with the breakdown of elastic components and airway distention is accomplished through stretch of the nonelastic structural components.
Collapsibility of the airway is determined by the contribution of airway cartilage and smooth muscle. Studies of the trachea in preterm lambs demonstrate that the preterm trachea is responsive to ACh by becoming stiffer, less deformable organs. However the magnitude of compliance and resistance changes consequent to smooth muscle contraction is small when compared to the full term and adult trachea suggesting a limited influence of airway smooth muscle on airway function in the preterm trachea. The mechanical properties (both tensile and compressive) of airway cartilage and smooth muscle are determined by the material composition of each tissue. Alterations in tissue composition with development may therefore explain differences in airway compliance. Proteoglycans are important constituents of the cartilaginous matrix and are known to confer rigidity to cartilage. Histochemical analysis by Bucher and Reid [17] has shown that the cartilaginous matrix of proximal human airways stains more basophilic near full term compared with a 16 weeks gestational age, suggesting increasing amounts of chondroitin sulfates with development. Considered collectively, these studies suggest that a relative lack of proteoglycans in immature airway cartilage may contribute to the collapsible nature of preterm lamb tracheae demonstrated in the present study. Additional factors that may contribute to developmental changes in the forcefulness of contraction of airway and non-airway smooth muscle in the preterm infant when compared to the full term and adult trachea includes:
1.
2.
Developmental changes in orientation of smooth muscle cells in the airway vessels from circular to oblique relative to the long axis of the vessel wall [58].
3.
Developmental changes in synthetic activity of vascular smooth muscle cells from primarily secretory (producing the extracellular proteins collagen and elastin) during the neonatal period to primarily contractile (producing the intracellular protein actomyosin) after 4 weeks of age in rats [59].
4.
Developmental changes in shape and function of airway smooth muscle cells (length and thickness doubled with age, although velocities of shortening were similar, in lambs vs. sheep) [60].
Forced expiration in the intact lung is typically associated with dynamic compression of the central airways, high resistance to airflow, and the establishment of a “flow-limiting segment” (FLS) of airway. Several studies have emphasized the importance of the compliance of the FLS in determining expiratory flow rate and have demonstrated how experimentally increasing or decreasing FLS compliance may respectively decrease [61–63] or increase [62] maximum expiratory flow. Accordingly, preterm infants with highly compliant airways might be expected to exhibit relatively greater airway compression, higher resistance, and severe flow limitation, which may exacerbate problems of gas exchange and breathing common in preterm infants. In the preterm trachea, relatively small compressive pressures are required to create significant airway compression and elevated resistance. The intrathoracic airways possess similar properties and are subject to similar dynamic compression at low efforts and flow limitation demonstrated in the preterm trachea [64] and as a consequence expiratory flow reserve is small. Complications associated with prematurity such as BPD [65–67] or the effects of mechanical ventilation [7, 8] may serve to further limit the preterm infant’s physiological range of breathing [66] and exacerbate problems of clinical management. Reflex constriction of airway smooth muscle plays an important physiological role during forced expiration, serving to stiffen the central airways and improve conditions for flow [68]. This mechanism might be particularly useful to the premature infant whose airways are extremely compliant; however, studies have suggested that the effect of ACh on airway mechanics is age dependent and that the ability of airway smooth muscle to decrease airway compliance and increase expiratory flow in the intact lung may be limited in the preterm trachea. As a result, reflex constriction of the airway smooth muscle results in relatively small changes in airway circumference in the preterm infant, and this lack of effectiveness may be a contributing factor to the relatively severe flow limitation observed in preterm infants.
The airway epithelium plays an important role in the modulation of smooth muscle function. Studies of adult airways have shown that airway epithelium generates relaxant and contractile factors that modulate the tone of the underlying smooth muscle [69–72]. Epithelial damage has also been associated with bronchial hyper-reactivity [73] as de-epithelialized smooth muscle cells exhibit greater force of contraction in response to acetylcholine stimulation than did a strip of tracheal smooth muscle with epithelium intact [38]. The magnitude of the effect was as great in the preterm as adult trachea, demonstrating that even during late gestation, epithelial integrity may be an important determinant of smooth muscle function, bronchial hyper-reactivity, and bronchodilator responsiveness.
When one assesses the effect of mechanical ventilation of airway structure and function the effect on the proximal and distal airways may be quite distinct. Histological studies of ventilated neonatal human and animal lungs found that, in addition to tracheomegaly, the peripheral airways were wider in lungs of neonates and animals that had been mechanically ventilated. The mechanisms responsible for this difference are not known, but may include distal/proximal (regional) airway differences, age-related differences in amount of cartilage, orientation of muscle fibers, forcefulness of contraction of muscle fibers, and receptor sensitivity of airway smooth muscle fibers. These differences would lead to mechanical ventilation affecting the more compliant distal and less compliant proximal airways differently.
Clinical Presentation
Improved care of premature infants in the NICUs has led to fewer rate and days of intubation and mechanical ventilation. In infants who required prolonged intubation, failed extubation is often the first clue of developing acquired airway problems. Suspicion of upper airway obstruction should be higher when an infant with minimum ventilator support develops significant respiratory distress soon after extubation. Studies such as direct laryngoscopy and bronchoscopy (DLB) can uncover findings of granuloma formation at infraglottic surfaces and grade 2 membranous SGS in a premature infant who failed multiple extubation attempts (Fig. 3). In a retrospective study, Pereira et al. reviewed 63 infants who underwent DLB for failed extubation between 1998 and 2006. In this study, more than 90 % of these infants had abnormal airway findings at the time of DLB with 39.7 % had either subglottic edema or edema. Infant who were born at 30 weeks GA or less, low birth weight and with history of chronic lung disease were twice likely to have these findings. In addition, the group of infants who required tracheostomy had double the number of days of ventilation, failed extubations, and incidence of subglottic compromise as compared with the group of infants who did not require tracheostomy [74].
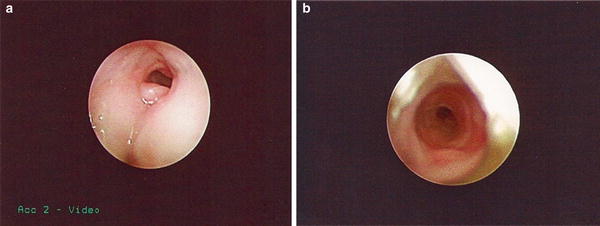
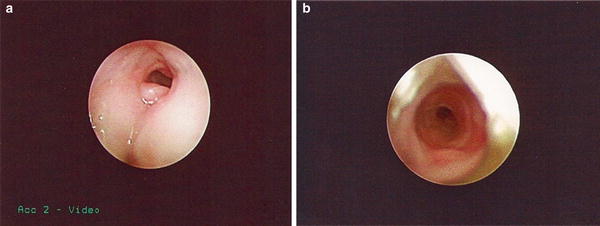
Fig. 3
Multiple failed extubation due to airway obstruction in an infant was born at 26 weeks gestation, receiving mechanical ventilation for 11 weeks. Bronchoscopy was performed at 37 weeks corrected age after multiple failed extubation attempts and showed (a) infraglottic granuloma formation; (b) membranous subglottic stenosis. Courtesy of Steve Sobol, (CHOP)
Stridor usually indicates an obstruction at the laryngotracheal airway. It may be inspiratory, expiratory or biphasic depending on the location of the obstruction: supraglottic lesions usually present with a coarse, inspiratory stridor, glottis and subglottic lesions often have stridor that described as musical and biphasic, whereas a pure tracheal lesion frequently produces expiratory stridor with a prolonged expiratory phase. However stridor is not always present in young infants with airway disorder. Some infants present with a weak, aphonic, or hoarse cry instead. Other infants may present with coughing and desaturation during feeds. In these cases, stridor may be intermittent and only become obvious or worse when the infant is agitated, crying, or during feeds (Fig. 4).
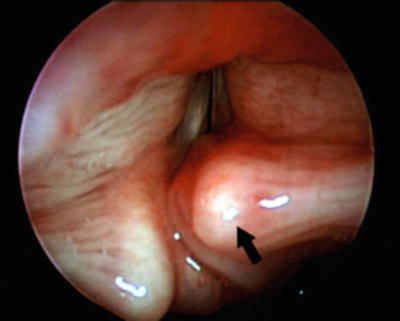
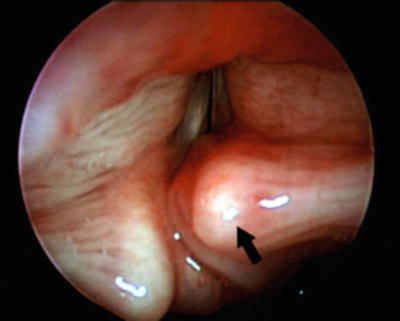
Fig. 4
Saccular cyst with prolapsed arytenoid causing airway obstruction with stridor. Courtesy of Luv Javia, (CHOP)
Patients with known BPD often exhibit episodic cyanotic spells known as “BPD” or “blue” spells. The etiology of these spells is often attributed to the exacerbated ventilation/perfusion (V/Q) mismatch during periods of agitation or pulmonary hypertension crisis. However, the “BPD” spells in some patients are actually caused by airway collapse due to severe TBM. During these episodes, the infants often have severe desaturation with bradycardia requiring PPV with high pressures. In non-intubated patients, signs of TBM may include coughing, respiratory distress with or without stridor, or episodes of apnea/bradycardia/desaturation (A/B/D). These A/B/D events in premature infants may be hard to differentiate from the events caused by apnea of prematurity. In addition to the cyanotic spells, TBM in infants with BPD can present with other nonspecific signs that are considered classic signs of BPD. These may include worsening hypoxia, hypercarbia, increasing oxygen requirement, increasing need for sedation, or simply as inability to wean respiratory support (Fig. 5). Often severe TBM in an infant may present as repeated inability to wean from positive pressure sometimes leading to progressively worsening pulmonary hypertension. In contrast to the diffuse, high-pitched and musical wheeze in asthma, wheeze in TBM is typically expiratory, central, low pitched and homophonous in nature [75] and mostly intermittent that worse during feeds and agitation.
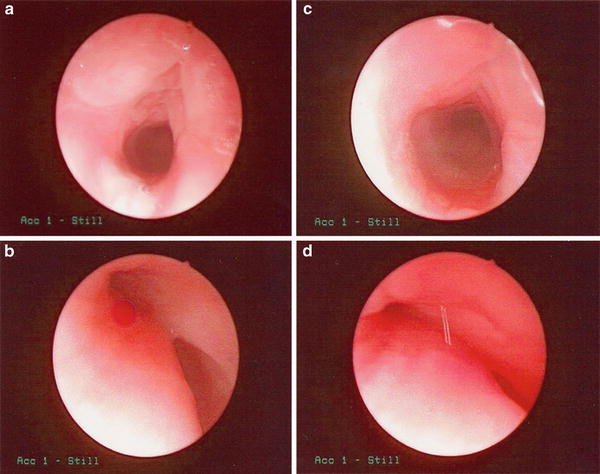
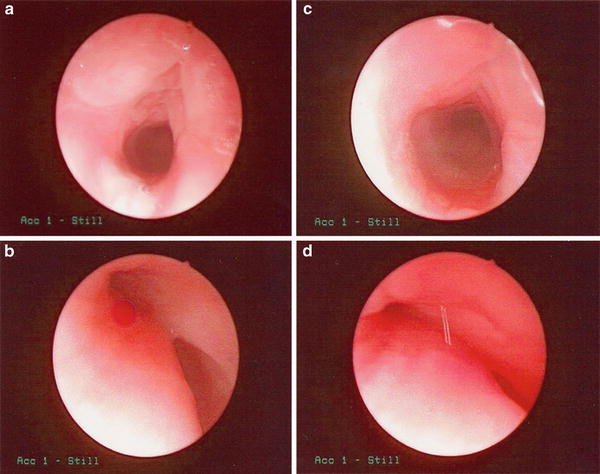
Fig. 5
Born at 23 weeks infant developed chronic respiratory failure and pulmonary hypertension due to severe airway malacia. (a & b): Bronchoscopy showing open trachea and bilateral bronchi during inspiration. (c & d): severe tracheal and bronchial collapse during expiration
Evaluation and Diagnosis
The diagnosis of acquired airway disorders in premature infants is difficult due to the small caliber of the airway, the rapid respiratory rate, and intolerance to various diagnostic procedures. When choosing diagnostic methods, issues such as reliability, the need for intubation, radiation dose, and contrast administration need to be considered.
Pulmonary Function Measures
Dynamic pulmonary compliance can be measured using an esophageal balloon and pneumotachometry. In infants who later develop chronic lung disease, compliance is significantly lower when compared with infants who recover uneventfully from neonatal respiratory distress. In addition to reflecting the elastic properties of the lung to some degree, the dynamic pulmonary compliance time-constant serves to indicate how equally various regions of the tracheobronchial tree are being ventilated. A high value for dynamic compliance in an infant with chronic lung disease probably indicates some degree of airway obstruction. Quasi-static methods of measuring respiratory system compliance in infants with BPD have verified that alterations in the elastic properties of the lung contribute significantly to the lower compliance values measured in these lungs. Static and dynamic compliance measurements have not been compared in this group of infants, so it is not clear whether the improvement in dynamic compliance seen in infants with moderate to severe BPD studied longitudinally represents a change in the characteristics of the lung parenchyma, a decrease in respiratory rate, or improvement in airway obstruction. Interestingly, both static and dynamic measurements of compliance have predictive value regarding the development of BPD in mechanically ventilated preterm infants [76, 77].
Resistance measurements, include airway resistance and is determined by plethysmography, pulmonary resistance measured by the esophageal balloon and pneumotachometry technique, or respiratory system resistance determined by the airway occlusion technique, are significantly elevated in infants with BPD [78–82]. However, when measurements of resistance or its reciprocal, conductance, have been made serially, values have approached normal by age 2 or 3 years [78, 79]. These developmental improvements in resistance values can reflect either resolution of airway obstruction or an increase in airway diameter related to growth. Specific conductance corrects for changes in resistance or conductance without the confounding variable of growth (lung size). Specific conductance is defined as conductance divided by lung volume at forced reserve capacity (FRC). Elevated specific conductance confirms the presence of airway obstruction in infants with BPD [78, 83].
Forced expiratory flow (FEF) measurements can be obtained using either the rapid thoracic compression technique [66, 81, 84] or the rapid deflation technique [85, 86]. Measurements with either technique better reflect flow in small airways and demonstrate significant small airways obstruction in infants with BPD. Longitudinal assessments of (FEF) demonstrate that infants with BPD have significant airway obstruction that does not resolve completely with growth. Maximal expiratory flow-volume (MEFV) demonstrates severe small airway obstruction as determined by a marked reduction in VmaxFRC. Among patients with moderate BPD who were weaned from mechanical ventilation before 5 months of age, VmaxFRC increased with increasing age to approximately 40 % of predicted value by 3 years of age [86]. In contrast, patients who required extended periods (10 months) of mechanical ventilation showed increase in Vmax25 over the same time period. Partial expiratory flow-volume (PEFV) curves generated over the tidal range of breathing showed that young infants with BPD had lower maximal flow at FRC (VmaxFRC) than young infants without BPD. Furthermore, the separation between tidal and forced-flow curves, which was interpreted as a measure of expiratory flow reserve [66], indicated that infants with BPD had a smaller expiratory flow reserve compared with normal infants. The FEF rates in infants with BPD were only half those of the normal infants and had not increased by 14.5–22 months of age [66]. These findings suggest that early exposure to PPV and high concentrations of oxygen not only caused early airway damage but also interfered with subsequent normal airway growth. Values of VmaxFRC have been used to estimate ventilation of small airways. However, studies have shown that collapse of central airways (i.e., tracheomalacia or bronchomalacia) can cause a marked reduction in values of VmaxFRC. When FEF measurements demonstrate marked reductions in VmaxFRC, repeating the measurement in the presence of CPAP is indicated to confirm if the reduction is related to central airway collapse or small airway obstruction. Functionally these airway abnormalities have been shown to persist in children and young adults with a history of BPD [87, 88].
Abnormalities in both tidal mechanics and FEF measurements confirm that airway reactivity is increased in infants with BPD. Taking this into consideration, airway reactivity testing becomes key in the evaluation and management of small airways disease in BPD. Acute decreases in pulmonary respiratory system and airway resistance have been reported in response to a variety of agonist and anticholinergic drugs [80, 81, 89–93]. The magnitude of this response was even more dramatic when forced flows were tested. Several other studies have confirmed airway reactivity in response to bronchodilator therapy.
Chest X-Ray
Chest X-ray (CXR) is the most widely used radiographic tool in the assessment of respiratory issues in premature infants. Many infants on mechanical ventilation require frequent CXR, sometimes daily, assessments to evaluate ETT position and lung expansion. Careful examine of these chest radiographs may provide important clue in the diagnosis of acquired airway anomalies in these young infants. Bhutani et al. reported roentgenographic appearance of acquired tracheomegaly in VLEW infants who required mechanical ventilation. They reported 91 % greater tracheal volume in the ventilated group as compared to weight-matched non-ventilated controls [36].
Some authors have suggested using combined inspiratory and expiratory plain radiographs for the diagnosis of TBM and reported sensitivity as high as 62 % when compared to microlaryngoscopy and bronchoscopy [94]. However, good inspiratory and expiratory films may be difficult to obtain in a premature infant with rapid respiratory rate. Nonetheless, significant segmental hyperinflation (Fig. 6) or air trapping (Fig. 7) on the plain CXR should raise the suspicion for TBM.
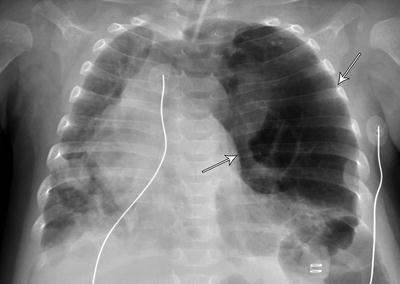
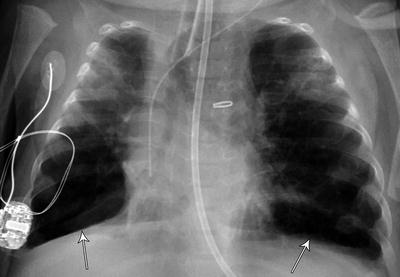
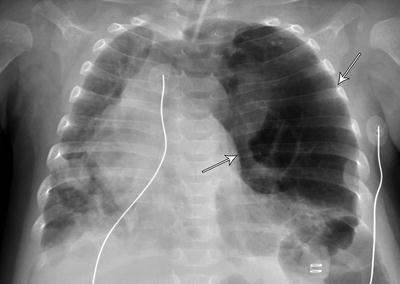
Fig. 6
Acquired lobar emphysema. Severe segmental hyperinflation in a premature infant requiring prolonged mechanical ventilation
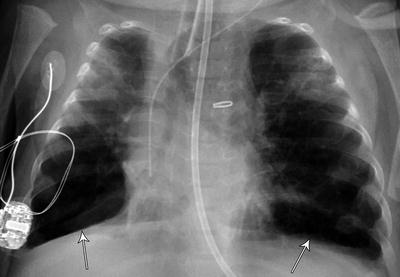
Fig. 7
Severe air trapping from severe tracheobronchial malacia due to persistent respiratory failure with oxygen requirement of 100 %
Fluoroscopy and Bronchography
Both fluoroscopy and bronchography have been used to diagnose TBM. However both methods have significant limitation such as radiation exposure, difficulty fully visualizing the airway especially in the lateral plane, underestimating the degree of tracheal collapse, and difficult to perform in a small premature infant with borderline respiratory reserve and small airways [95]. Sanchez et al. compared fluoroscopy with flexible bronchoscopy in 22 children at 1 month to 8 years of age. They found that airway fluoroscopy was poorly sensitive (23.8 %) but highly specific (100 %) in the diagnosis of tracheomalacia [96].
CT
CT is a noninvasive method that enables precise and objective delineation of the location, extent, and depth of tracheobronchial pathology (Fig. 8). When compared to bronchoscopy, concordant rate as high as 83–100 % has been reported in the diagnosis of TBM. Figure 9 shows significantly decreased tracheal size during expiration phase in an infant with severe bronchomalacia. In addition, evaluation of the adjacent cardiovascular system and lung parenchyma can be simultaneously obtained, especially when combined with CT angiography (CTA) (Figs. 10, 11, and 12). In recent years, newer CT techniques such as dynamic, volumetric imaging using wide-range multi-detector CT have increased the sensitivity and accuracy in the diagnosis of airway stenosis and malacia. These techniques have been successfully used in young infants [97, 98]. Although CT is becoming increasingly popular as a diagnostic tool for the noninvasive evaluation of respiratory tract disorders in pediatric patients, attention needs to be paid to reduce the exposure to radiation. This requires each scan to be carefully planned in accordance to body size, respiratory rate, cooperation, and IV access. Using a standardized dose reduction protocol, radiation dose from a CTA has been reduced from 3.2 mSv in 2007 to the current level of 0.2–0.4 mSV at the Children’s Hospital of Philadelphia.
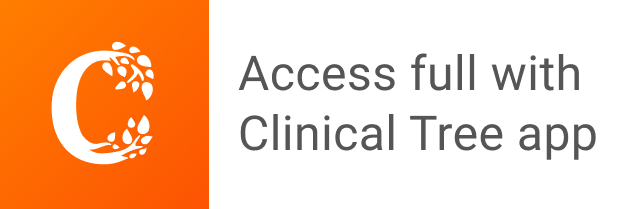