Fig. 8.1
Morphological changes in the optic nerve head with progression of myopia. (a) Fundus photograph of a myopic eye in a 14-year-old male patient. (b) Fundus photograph of the same eye when the patient was 19 years old. The enlargement of the area of crescent peripapillary atrophy (white arrow), straightening of the vessels, and distinctly visible choroidal vessels (tigroid fundus) are evident. The origin of the retinal artery is obscured by the rim because of the steeply tilted disc (black arrow)
8.3 Systemic Blood Flow and Glaucoma
Newman-Casey et al. reported that hypertension (hazard ratio, HR: 1.17) and diabetes (HR: 1.35) were risk factors for OAG in a large cohort epidemiological study that ran from 2001 to 2007 [13]. Their findings have since been reinforced [14–17], although the identification of conditions associated with metabolic syndrome, such as hypertension and diabetes, as risk factors for glaucoma remains controversial [18–20]. Additionally, low systemic blood pressure has been reported to be a major risk factor for glaucoma [4, 19, 17]. Individuals who experience nightly dips in systemic blood pressure and fluctuation in systemic blood pressure are thought to be particularly vulnerable [21–23]. These irregularities in blood pressure can result in low ocular perfusion pressure and insufficient ocular blood supply [24, 25].
8.4 Ocular Blood Supply
The ophthalmic artery is the major vascular vessel providing blood to the inner retina and optic nerve. The central retinal artery, which branches off the ophthalmic artery and enters the optic nerve approximately 12 mm posterior to the globe, supplies blood to the retinal ganglion cell bodies in the inner retina and nerve fibers. The central retinal artery also provides partial perfusion to the superficial optic disc. The pre-laminar region and lamina cribrosa are mainly supplied from branches of the posterior ciliary arteries (PCA), branching off from the ophthalmic artery and also from other vessels originating from the arterial circle of Zinn-Haller (ZHAC). In addition, fine centripetal branches from the peripapillary choroid provide blood to the pre-laminar region [26–28]. The blood supply in this region is sectorial in nature, similar to distribution of the PCA circulation. These branches from the PCA pierce the sclero-optic junction and enter the lamina cribrosa through the border tissue of Elschnig at the choroid [29]. It is generally assumed that microvessels throughout the optic nerve have characteristics of the blood-brain barrier (BBB), since tracers such as peroxidase do not pass out of the optic nerve capillaries [30]. However, a study of immunohistochemistry using non-BBB markers showed that microvessels in the pre-laminar region of the optic nerve head (ONH) lack typical BBB characteristics and display nonspecific permeability [31], possibly mediated by vesicular transport.
8.4.1 Devices to Measure Ocular Circulation
The identification of a relationship between ocular blood flow and the pathogenesis of glaucoma has inspired much recent research. Accurate methods to measure ocular blood flow are critical to these investigations, and various quantitative and qualitative techniques have been introduced to achieve the necessary accuracy [32, 33]. These include fluorescein angiography, laser or ultrasound Doppler shift, and devices based on the laser speckle phenomenon. These techniques each have characteristic advantages in measuring glaucoma-related tissues, such as the retinal vessels, optic nerve, short PCA, the ZHAC, ocular artery, and choroid, and their complementary use allows an overall understanding of ocular blood flow. Here, we provide an outline of the major techniques currently used in ophthalmological research to measure ocular blood flow.
8.4.1.1 Angiography
Fluorescein angiography allows for the visualization of morphological structures passing a fluorescent dye through them. The mean circulation time is used as a measure of blood velocity. However, the relationship between retinal blood flow and transit time in fluorescein angiography is weak [34]. Angiography can be used to evaluate the perfusion of microvascular beds and to identify the failure of vessels, which is indicated by hyperfluorescence, defects in fluorescence, and leakage [35]. A scanning laser ophthalmoscope can also be used to quantify macular blood flow velocity by tracking hyperfluorescent and hypofluorescent dots as they pass through the perifoveal capillaries [36]. This approach, does, however, require excellent image quality, because otherwise these hyperfluorescent and hypofluorescent dots cannot be identified unequivocally in consecutive images [32]. Angiography with indocyanin green (ICG) has a peak spectral absorption at about 800 nm and is often used to assess choroidal vessels and circulation. The major advantages of ICG-based methods for the assessment of choroidal blood flow are superior penetration of near infrared light into pigmented ocular structures and improved binding to plasma proteins, which prevents marked leakage from vessels to the surrounding tissue [33].
8.4.1.2 Laser Doppler Velocimetry
Laser Doppler velocimetry (LDV) is used to determine the velocity of blood cells in the larger retinal vessels [37]. This technique uses the optical Doppler shift of light, which is directly proportional to the velocity of blood cells. LDV combined with measurements of vessel diameter at the same site enables the determination of volumetric flow rate in the major retinal vessels. However, LDV cannot be used to measure ONH blood flow [33].
8.4.1.3 Color Doppler Imaging
Color Doppler imaging (CDI) is used to assess blood velocity, especially in the retrobulbar vessels, including the ophthalmic artery, central retinal artery, and short posterior ciliary arteries. CDI facilitates the determination of blood flow velocity by color-coding the Doppler frequency shift of ultrasonic waves and super imposing the resulting color map on a B-scan image [38]. The measurement parameters of CDI are peak systolic velocity (PSV), end diastolic velocity (EDV), and mean velocity. The resistive index (RI) can also be calculated with the following formula: RI = (PSV − EDV)/PSV. However, the usefulness of the RI as an indicator of vessel resistance in the central retinal artery remains controversial [39]. CDI produces highly reproducible measurements of blood velocity in the ophthalmic artery, but in narrower vessels, such as the short PCA, measurement values tend to fluctuate [40].
8.4.1.4 Laser Doppler Flowmetry
Laser Doppler flowmetry (LDF) is based on the scattering effect of light in tissue and can measure both relative blood velocity and blood flow. This technique uses a laser beam to illuminate a small volume of tissue that does contain any large visible vessels. Some of the light scattered by the tissue and moving red blood cells is then detected by a photo detector [41], and based on the scattering effect, relative measurements of the mean velocity of the erythrocytes and the volume of blood can be obtained. A relative value for blood flow can then be determined by calculating the product of the velocity and volume measurements. However, the tissues which have influence on the light scattering vary considerably between individuals. Therefore, when comparing interindividually, this character of LDF should be taken into account.
8.4.1.5 Laser Speckle Flowgraphy
Laser speckle flowgraphy (LSFG) uses the laser speckle phenomenon. This phenomenon occurs when surfaces are illuminated by coherent laser light. When the ocular fundus is illuminated by laser light, the scattering of the light by the tissue gives rise to a speckle pattern. Changes in the velocity of blood flow cause blurring of the speckle pattern, and this blurring can be quantified by specialized software. The main measurement parameter of LSFG is mean blur rate, an arbitrary unit calculated from the light intensity of the speckle pattern on a point-by-point basis.
8.4.1.6 Pulsatile Ocular Blood Flow
Pneumotonometric methods are used to estimate pulsatile ocular blood flow (POBF) on the basis of changes in measurement of IOP during the cardiac cycle [42]. This provides a non-invasive, continuous measurement of IOP, which, when compared to the rate of change of IOP, can be used to estimate the pulsatile component of ocular blood flow [43]. POBF analysis includes the determination of fundus pulsation amplitude and pulse amplitude. The main limitation of this technique arises from the lack of information it provides on the non-pulsatile component of ocular blood flow [32].
8.5 Ocular Circulation as a Risk Factor for Glaucoma
Population-based studies have revealed that low blood pressure and low ocular perfusion pressure (OPP) increase the prevalence of glaucoma. Adequate irrigation of ocular tissues can only be ensured by adequate OPP, which depends on a complex regulation process to balance BP and IOP. The vascular hypothesis of glaucoma pathogenesis is thus based on the premise that abnormal perfusion, with the subsequent deterioration in ONH circulation, plays a major role in the pathophysiology of damage in glaucoma. Many studies performed with a variety of techniques have found that glaucoma is associated with altered ocular blood flow, particularly ocular blood flow in the posterior pole of the eye [44–48]. In eyes with glaucomatous visual field deterioration, retrobulbar blood velocity decreases significantly, and the resistance of the vessels increases significantly [45–49]. In severe glaucoma, deterioration of ocular circulation also increases with the progression of glaucoma and visual field loss [50]. In glaucoma patients with asymmetric visual field loss, the more affected eye also has lower blood velocity in the central retinal artery than the less affected eye [51]. CDI data shows that retrobulbar blood velocity in eyes with glaucoma is significantly correlated with mean blood pressure [52]. In comparison, healthy eyes show a lower, although still significant, correlation. This implies that the vascular autoregulation system in ocular arteries, which acts in response to changes in perfusion pressure [53], is compromised in eyes with glaucoma. LSFG studies of pre-laminar tissue in the ONH of eyes with glaucoma have shown that blood flow in this area is significantly lower than normal and that it correlates to the severity of glaucoma. This is consistent with histological studies, indicating that dropout of the capillaries within the pre-laminar and laminar lesions in the ONH may be occurring.
8.6 Ocular Circulation Studies in Myopia
In myopic eyes, elongation of the axial length leads to stretching of the sclera, and the optic disc becoming elliptical and tilted temporally, changes which are associated with enlarged crescent PPA [54]. Temporal crescent PPA is a common consequence of scleral stretching [55]. Furthermore, there is evidence that myopia leads to the deterioration of ocular circulation. With myopic change, the diameter of the retinal arteries becomes smaller and retinal blood flow in the retinal arteries decreases [56–58]. Retrobulbar blood flow and choroidal circulation also decrease in severe myopia [58, 59].
This leads to the question of whether there is an association between glaucoma and myopic changes in ocular circulation. Nicolela’s classification system of ONH morphology categorizes elliptical ONHs with temporal crescent PPA as the “myopic glaucomatous type” [60, 61]. Myopic eyes with glaucoma tend to significantly younger at the time of diagnosis, with the central vision frequently threatened by glaucomatous scotoma. There are also a higher number of patients of Asian origin. Moreover, in the four glaucomatous optic disc types, retrobulbar circulation in the myopic glaucomatous type shows lower PSV, determined by CDI, in the ophthalmic artery than the focal ischemic type [62]. In the LSFG, tissue circulation in the optic nerve area in myopic glaucoma has also been reported to decrease, in correlation with visual field defects [63]. Figure 8.2 shows the difference in blood circulation between a glaucomatous ONH with myopic deformation and one with focal rim notch. Changes in ocular circulation in myopic eyes thus appear to be one of the mechanisms of glaucoma pathogenesis.
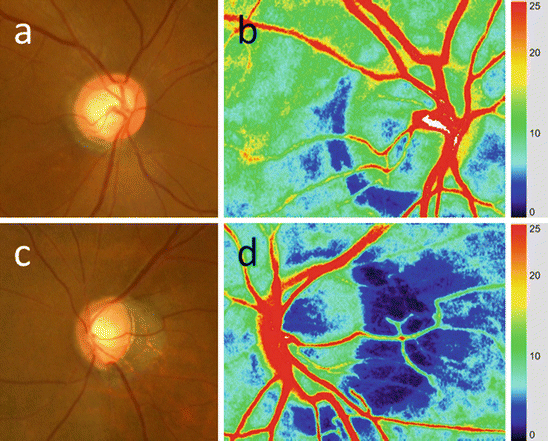
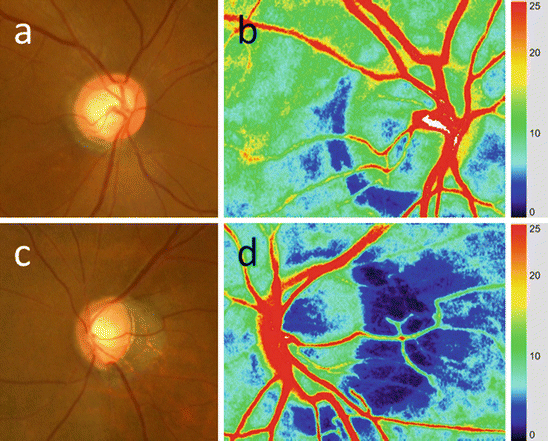
Fig. 8.2
(a) Blood flow in optic nerve head of a patient with asymmetric refractive error (color-coded map, laser speckle flowgraphy: LSFG). (b) Fundus photograph of right eye with normal refractive error (0.15 diopters). (c) Color-coded LSFG map of right eye. Warmer colors indicate higher mean blur rate (MBR: arbitrary unit used by LSFG). (d) Fundus photograph of left eye with high refractive error (−3.38 diopters). (e) Color-coded LSFG map of left eye. The proportion of warm colors in the optic nerve head is lower than in the right eye (b)
8.6.1 Retinal Circulation in Myopic Eyes
Quigley and Cohen showed that perfusion pressure in the retinal artery becomes attenuated with elongation of the axial length, a finding that arose from the observation that the frequency of diabetic retinopathy is lower in myopic eyes than emmetropic eyes [64]. The attenuation of perfusion pressure in arteriole segments is measured by the pressure attenuation index (PAI), which is proportional to the length and inversely proportional to the diameter of the segment. There is a positive correlation between PAI and axial length. The protective effect of myopia against diabetic retinopathy thus seems to arise from axial elongation causing pressure attenuation in the retinal artery. However, decreased perfusion pressure in the retinal arteries may also eventually lead to glaucomatous damage, because the retinal artery feeds the inner retina, including the ganglion cells and their axons [65, 66]. In addition, as myopia progresses, the diameter of the retinal arteries becomes smaller [57]. Measurement of retinal arterial velocity and the diameter of major superotemporal or inferotemporal arteries indicate that retinal blood flow decreases in high myopia, mainly due to the narrowing of the retinal vessel diameter [56]. This structural change in myopia may have a negative effect on retinal irrigation.
8.6.2 Hemodynamics of the Choroid in Myopia
Choroidal circulation, especially in the peripapillary area, is important to the study of POAG because the blood supply of the pre-laminar section of the ONH depends on the peripapillary choroid [67]. The elongated axial length of highly myopic eyes causes stretching of the sclera between the disc and the posterior pole, often leading to thinning of the peripapillary choroid. Although it is difficult to directly measure choroidal circulation, choroidal thinning is thought to indicate reduced choroidal circulation [68]. Disturbances in choroidal circulation and stretching of the sclera in highly myopic eyes are also thought to be associated with enlarged areas of peripapillary atrophy [69]. The vascular/hemodynamic theory may also help in understanding choroidal hemodynamics in myopia, as it describes glaucomatous optic neuropathy as an ischemic injury resulting in reduced ocular blood flow at the level of the lamina cribrosa [70].
A number of studies have investigated the association between choroidal hemodynamics and the pathogenesis of glaucoma. Histologic studies have found only an inconsistent association between glaucoma and choroidal thickness [71]. In vivo studies of choroidal thickness with optical coherence tomography (OCT) have also had conflicting results. Hirooka et al. reported that peripapillary choroidal thickness was associated with glaucoma, and Usui et al. reported that highly myopic eyes with normal tension glaucoma had greater thinning of the choroid than controls matched for age, spherical equivalent refractive error, and axial length [72]. In contrast, Mwanza et al. reported that there was no association between glaucoma and choroidal thickness, in a study that used enhanced-depth imaging techniques and spectral domain OCT [73]. These conflicting results may be due to differences between the studies in measurement location, the distribution of subjects, and the incidence in the subjects of different types of glaucoma, especially myopic glaucoma. In addition, despite the considerable improvements in ocular posterior segment imaging offered by OCT, in vivo assessment of the choroid remains difficult. Data in support of the association between choroidal blood flow and glaucoma is also available from an eye bank histological study showing that eyes with advanced glaucoma have lower capillary density in the choriocapillaris of the macula, temporal peripapillary, and equatorial choroid than control eyes [74]. This may reflect the fact that the pre-laminar region of the ONH is fed by the peripapillary choroid [26–28].
8.6.3 Circulation in the Posterior Ciliary Arteries and the Arterial Circle of Zinn-Haller in Myopic Eyes
The PCAs are the main vessels supplying the ONH and are particularly important sources of circulation supplying the pre-laminar tissue and lamina cribrosa. The status of the PCAs is thus an important indicator of blood flow in glaucoma. CDI data shows that blood velocity in the PCAs is decreased in highly myopic eyes [58]. The occlusion of the para-optic branches of the short PCAs could induce time-dependent deterioration in the area of anoxic segmental degeneration. Thus, the circulation of PCAs is important for understanding the pathogenesis of glaucoma [75].
The peripapillary ZHAC which is fed by the PCAs branches into the intra-laminar region of the ONH and is the major arterial contributor to the vascular system in the lamina cribrosa [28, 76]. In high myopia, the ZHAC is located within the myopic crescent, with the vessels appearing as a hyporeflective circle within the peripapillary sclera. The ZHAC usually has a rhomboid configuration [77].
In a histomorphometric study, the distance of the ZHAC from the peripapillary ring (located at the optic disc border) was found to increase significantly with axial elongation and other related parameters [78, 79]. The distance of the ZHAC from the lamina cribrosa, for which it is the main source of arterial blood, would thus also increase. Although the relationship of alterations to the ZHAC caused by axial elongation and glaucoma is thus not yet fully understood, it is possible that these changes affect the blood supply to the ONH and could serve as an indicator of the onset of glaucoma.
8.7 Impact of Ischemia on Retinal Ganglion Cells
The results of many studies show that ischemia has multiple detrimental effects on the retinal ganglion cells (RGCs) and their axons [80–82]. Mechanisms implicated in RGC death include hypoxia-induced reactive oxygen species (ROS) [83], excitotoxicity, nitric oxide (NO) [84, 85], and the inflammation reaction [86]. There is strong evidence that chronic retinal ischemia also results in a variety of harmful intra-retinal events.
Ischemia and reperfusion induce ROS generation in three phases: in the first phase, mitochondria generate ROS; in the second phase, xanthine oxidase is activated; and in the third phase, Ca2+- dependent ROS generation begins [87]. Investigations of ischemia in RGCs have included ROS generation and cytotoxicity [83] and NO [88]. NO, synthesized by nitric oxide synthase (NOS), has been shown to have neuroprotective and neurotoxic roles [89], and some experiments have shown that NO production contributes to cytotoxicity resulting in cell death [84, 85]. Excitatory amino acids have been also reported to play an important role in the development of hypoxic-ischemic retinal injury [90, 91]. Glutamate excitotoxicity may also facilitate primary and/or secondary degeneration of the RGCs in glaucoma [92, 93].
Ischemia causes increased permeability of the blood-retinal barrier due to vascular endothelial growth factor, NO, free radicals, and aquaporin-4 protein expression resulting in serum leakage into retinal tissue [94]. The compression induced by tissue edema is thought to occur secondarily and to also be a cause of RGC degeneration [95].
8.8 Conclusion
Glaucoma is a multifactorial disease with a pathogenesis that remains unclear. However, myopia is known to be a major risk factor for glaucoma, and an understanding of myopia’s role in glaucoma is important. A number of studies have reported that there is an association between ocular circulation deterioration and myopic morphological change. Elucidation of the effect of myopia on ocular circulation might lead to improved knowledge of the pathogenesis of myopic glaucoma (Fig. 8.3, the summary of this chapter).
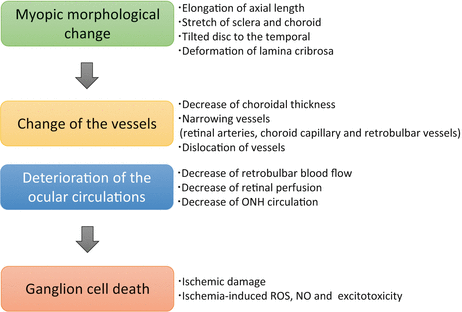
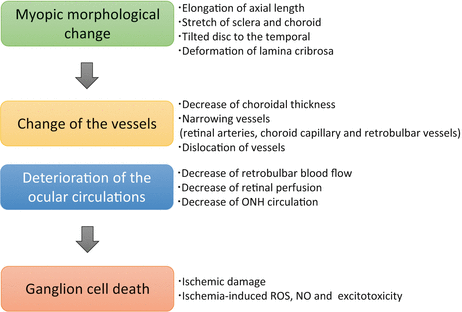
Fig. 8.3
Summary of this chapter: Ocular blood flow in myopic glaucoma
References
1.
Quigley HA, Broman AT (2006) The number of people with glaucoma worldwide in 2010 and 2020. Br J Ophthalmol 90(3):262–267. doi:90/3/262 [pii] 10.1136/bjo.2005.081224 PubMedCentralCrossRefPubMed
2.
Kass MA, Heuer DK, Higginbotham EJ, Johnson CA, Keltner JL, Miller JP, Parrish RK 2nd, Wilson MR, Gordon MO (2002) The Ocular Hypertension Treatment Study: a randomized trial determines that topical ocular hypotensive medication delays or prevents the onset of primary open-angle glaucoma. Arch Ophthalmol 120(6):701–713; discussion 829–730. doi: ecs20045 [pii]CrossRefPubMed
3.
Comparison of glaucomatous progression between untreated patients with normal-tension glaucoma and patients with therapeutically reduced intraocular pressures. Collaborative Normal-Tension Glaucoma Study Group (1998). Am J Ophthalmol 126 (4):487–497. doi:S0002939498002232 [pii]
4.
Leske MC, Heijl A, Hyman L, Bengtsson B, Dong L, Yang Z (2007) Predictors of long-term progression in the early manifest glaucoma trial. Ophthalmology 114(11):1965–1972. doi:S0161-6420(07)00241-2 [pii] 10.1016/j.ophtha.2007.03.016 CrossRefPubMed
5.
Phelps CD, Corbett JJ (1985) Migraine and low-tension glaucoma. A case-control study. Invest Ophthalmol Vis Sci 26(8):1105–1108PubMed
6.
Sommer A, Tielsch JM, Katz J, Quigley HA, Gottsch JD, Javitt JC, Martone JF, Royall RM, Witt KA, Ezrine S (1991) Racial differences in the cause-specific prevalence of blindness in east Baltimore. N Engl J Med 325(20):1412–1417. doi:10.1056/NEJM199111143252004 CrossRefPubMed
7.
Mitchell P, Hourihan F, Sandbach J, Wang JJ (1999) The relationship between glaucoma and myopia: the Blue Mountains Eye Study. Ophthalmology 106(10):2010–2015CrossRefPubMed
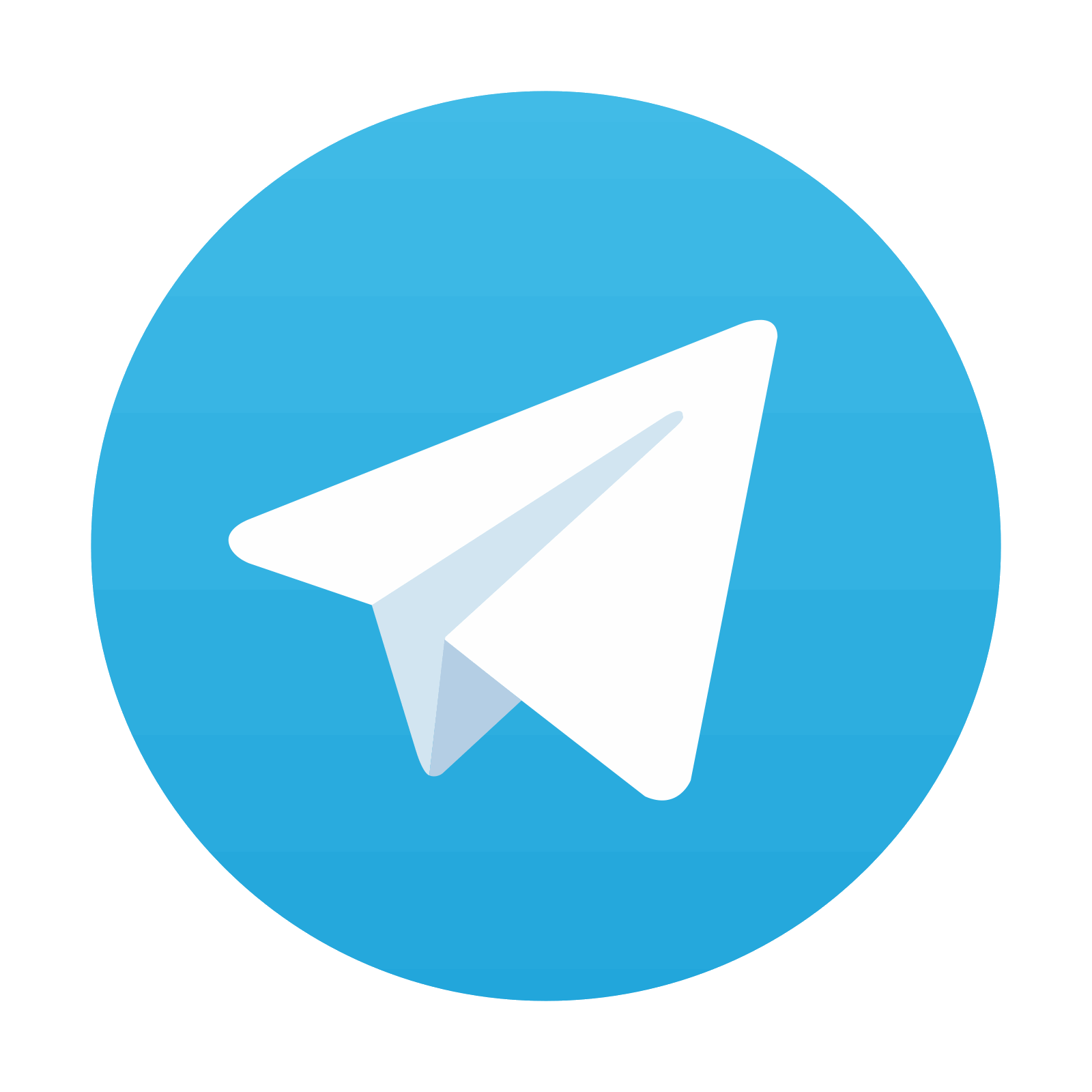
Stay updated, free articles. Join our Telegram channel
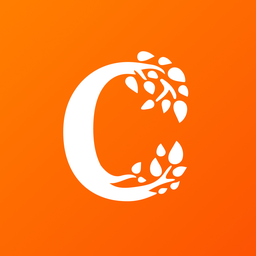
Full access? Get Clinical Tree
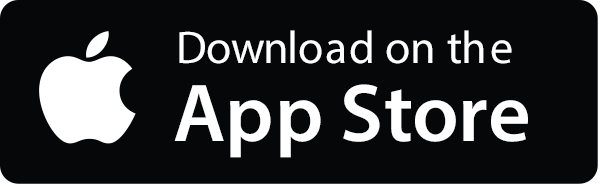
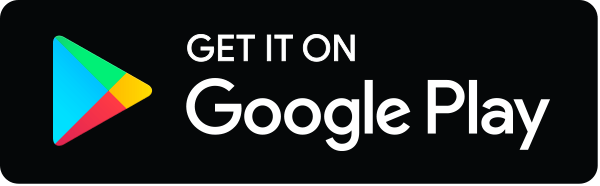