Metabolism and Photochemistry of The Retina
Muayyad R. Al-Ubaidi
Robert E. Anderson
David M. Sherry
The retina is a thin, delicate, transparent tissue that lines the posterior of the eye and is situated between the vitreous humor and the retinal pigment epithelium (RPE) (Fig. 12-1A). The inner limiting membrane, which separates the retina from the vitreous humor, is composed of the foot processes of the glial cell, the Müller cell. This is the only cell that traverses the entire retinal expanse; the distal ends of the Müller cell form the outer limiting membrane between the cell bodies of the photoreceptor cells. Three distinct cell layers are evident in Figure 12-1. The most distal one contains the photoreceptor cells, the rods, and cones. Rods are used for night vision and are most abundant in the peripheral retina. Cones are located in the central region of the retina, the macula, and are responsible for color perception and visual acuity. The nuclei of rod and cone cells make up the outer nuclear layer (ONL). Both rods and cones make synaptic contact in the outer plexiform layer (OPL) with horizontal cells and bipolar cells. The nuclei of the horizontal and bipolar cells make up the inner nuclear layer (INL). Bipolar cells are the second-order neurons of the retina and transfer the visual message generated in the photoreceptors to the ganglion cells, the third-order neurons. Amacrine and interphotoreceptor cells also make synaptic contact in the OPL with ganglion and bipolar cells, as well as with each other. The visual message exits the eye by way of the ganglion cell axons, which collectively form the optic nerve.
The function of the retina, to transduce light into an electrical signal that can be transmitted to the brain, is accomplished through the photolysis of light-sensitive visual pigments that are present in the outer segments (OS) of photoreceptor cells. A schematic of a photoreceptor cell, presented in Figure 12-2, illustrates the high degree of polarization of this structure. The proximal end of the photoreceptor cell is a nerve terminal, which makes chemical synapses with horizontal and bipolar cells. The inner segment (IS) contains the organelles, which are responsible for the metabolic activity of the cell. The biosynthesis of proteins and lipids occurs on the endoplasmic reticulum in the myoid region of the inner segment, and energy (e.g., adenosine triphosphate [ATP], guanosine triphosphate [GTP]) is generated in the mitochondria in the ellipsoid region of the inner segment. The IS is connected to the distal end of the photoreceptor cell, the OS, by a narrow connecting cilium. OSs occupy the subretinal space and are made up of stacks of membrane discs enclosed within a plasma membrane. The subretinal space is an organized network of fibers made up of complex proteoglycan macromolecules that surround the outer segments of both rod and cone cells. This fibrous network is called the interphotoreceptor matrix. The distal tips of the OSs are in intimate contact with epithelial processes of the retinal pigment epithelium (RPE). This contact is essential for the survival of photoreceptor cells. Disruption of the interphotoreceptor matrix or separation of the outer segments from the pigment epithelium leads to a retinal detachment.
Four types of photoreceptor cells are found in the human retina, and these are determined by the types of light-sensitive protein photopigments that are contained in their OS membranes. Each photopigment has a distinctive absorption spectrum. Rhodopsin is the photopigment of rod outer segments (ROS) and absorbs light maximally at 498 nm. There are three types of cone photoreceptor cells with each containing only one type of photopigment. The cone pigments are named for the color of light they absorb: blue (437 nm), green (533 nm), and red (564 nm). Cone photoreceptor cells are arranged in a mosaic pattern throughout the macula.
The OSs are made up of stacks of membranous discs surrounded by a plasma membrane (Fig. 12-2). The plasma membrane is continuous with the inner segment through the narrow connecting cilium. In ROS, these discs are free-floating, except for a few at the base, and are not continuous with each other or with the plasma membrane. Cone outer segment discs are not free-floating, so the plasma and disc membranes are continuous.
CHEMISTRY OF PHOTORECEPTOR OUTER SEGMENTS
The bulk of the chemical analyses on photoreceptor cell outer segments have been done on ROS, because rods are by far the most abundant of the photoreceptors. Large quantities of relatively pure ROS are easy to prepare. Isolated ROS contain both disc and plasma membranes; however, because the plasma membrane is only 1% to 2% of the total ROS, an analysis of ROS is essentially an analysis of disc membranes. Only in recent years have relatively pure plasma membranes been prepared in quantities large enough for analysis.
ROD DISC MEMBRANES
The chemical makeup of the disc membranes is relatively simple. The membrane structure is a bilayer; about half of the molecules are integral proteins, and the other half are lipids. Phospholipids make up about 90% of the disc membrane lipids. Phosphatidylcholine (40%–45%) and phosphatidylethanolamine (35%–40%) are the major phospholipids, but relatively large amounts of phosphatidylserine (10%–15%) are also found. Minor phospholipids (about 5%) include phosphatidylinositol (PI), phosphatidylinositol 4-phosphate (PIP), phosphatidylinositol 4,5-bisphosphate, and sphingomyelin. The cholesterol content of the discs is <10%, which is lower than is usually found in plasma membranes. Small amounts of free fatty acids and diacylglycerol are also found in disc membranes. An interesting feature of cholesterol in the discs is that the most basal discs have higher levels of cholesterol than those near the apical tips.1,2 There does not appear to be a similar gradation in phospholipid or fatty acid distribution. The fatty acids of ROS lipids are somewhat unique in that the major component is docosahexaenoic acid (22:6n-3), a long-chain polyunsaturated fatty acid with six double bonds.3 This fatty acid also occurs in large amounts in synaptic terminals in the central nervous system but is not found to any great extent in nonneural tissues.
ROS contain integral (part of the membrane), peripheral (associated with the membrane), and soluble proteins. The major integral protein in rod disc membranes is the visual pigment rhodopsin (approximately 90%), a glycolipoprotein of about 40,000 molecular weight. The interaction of rhodopsin in the disc membrane is shown schematically in Figure 12-3. Rhodopsin has seven α-helical loops that traverse the lipid bilayer.4 These are important in some of the interactions of rhodopsin with other ROS proteins. Its C-terminus is oriented toward the cytoplasmic surface of the disc, and its N-terminus, which contains two carbohydrate side chains, is oriented toward the intradisc space. The C-terminus contains six residues of serine and threonine, three of which are phosphorylation sites (serines 334, 338, and 343, Fig. 12-3).5,6 11-cis-retinal is the chromophore of rhodopsin and is linked chemically by a Schiff base to lysine-296. The loops of rhodopsin outside of the lipid bilayer are important in the binding of peripheral and soluble proteins following photobleaching. Two adjacent cysteine molecules near the C-terminus contain thioesters of palmitic acid, whose hydrophobic tails are embedded in the lipid bilayer7 anchoring the C-terminus to the disk membrane and creating a specialized surface region; a fourth cytoplasmic loop (amino acids 310–321).8,9 provides an additional region for protein-protein interaction. This anchoring of the C-terminus of rhodopsin is expected to cause conformational constraints.10 It was suggested that the fourth cytoplasmic domain created by the insertion of the palmitoyl groups into the membrane play a role in the binding and activation of transducin.
Several integral proteins are present in ROS in small amounts. Two are located at the edges of the discs near the plasma membrane. Peripherin (also called RDS, retinal degeneration slow), so named because it is located near the edges (rims) of the discs,11 is necessary for the development of the normal architecture of the outer segments. Also located near the rims of the disc is the ATP-binding cassette transporter ABCA4, which is thought to actively transport or flip N-retinylidene-phosphatidylethanolamine from the lumen to the cytoplasmic side of disc membranes following the photobleaching of rhodopsin. Mutations in peripherin/RDS lead to various retinal degeneration phenotypes, depending on the location of the mutation.12 Mutations in ABCA4 are the cause of autosomal recessive Stargardt disease.13
Nonintegral proteins of ROS include a cyclic guanosine monophosphate (cGMP)-specific phosphodiesterase, guanylate cyclase, arrestin (S antigen, 48 K protein), rhodopsin kinase, protein kinase C, other protein kinases, phosphoprotein phosphatase, phosducin, transducin, phospholipase C, alcohol dehydrogenase, glucose-6-phosphate dehydrogenase, glutathione peroxidase, glutathione reductase, diacylglycerol kinase, PI kinase, PIP kinase, recoverin, and others yet to be discovered. Several of the ROS proteins are directly involved in visual transduction and are discussed later in greater detail.
ROD PLASMA MEMBRANES
Because the discs are derived from the plasma membrane, one would think that the chemistry of the disc and plasma membranes would be the same. However, this is not the case. Studies have now shown that the ROS plasma membrane has a different lipid and protein composition.14,15 The major differences in the lipids are a larger amount of cholesterol and lower level of 22:6n-3 in the plasma membrane. Rhodopsin is present in the plasma membrane, although at a much lower level than in the discs. The plasma membrane contains the cyclic GMP-dependent sodium channel, an integral protein that controls the passive entry of sodium (and, to a lesser extent, calcium) into the outer segment in the dark. Calcium is extruded from the outer segment by a calcium-sodium exchanger in the plasma membrane, and sodium is extruded from the photoreceptor cell by ouabain-sensitive pumps located in the ellipsoid region of the inner segment. This passive influx of sodium in the dark is the dark current described by electrophysiologists.
CONE VISUAL PIGMENTS
All three cone pigments have been identified, and their amino acid sequence is known. All three share sequence homologies with rhodopsin, suggesting that all four visual pigments are derived from a common ancestral gene. Point mutations involving single amino acid substitutions as well as rearrangements in the cone pigment genes have been described in color-blind individuals.16,17 Thus, color blindness can result from a single point mutation in the DNA encoding one of the color pigments, leading to the synthesis of a nonfunctional visual protein. Alternatively, recombinational rearrangements in the red/green pigments genes can also lead to color blindness.17,18 These proteins either do not form a photolabile molecule with 11-cis retinal or do not activate transducin and therefore fail to activate the phosphodiesterase when photobleached. In some cases of color pigment genes rearrangements, a functional pigment that absorbs photons and initiates visual excitation can be generated. This is the so-called anomalous color blindness, which is identified psychophysically when the test subject does not match the “normal” spectral sensitivity patterns of human visual pigments.
Anomalies in color vision are not limited to mutations in the color pigment genes. Patients with a mutation (Pro50Leu) in the gene-encoding guanylate cyclase activator protein-1 (GCAP-1) exhibit decreased visual acuity and loss of color vision that occurs after the age of 20 years. Electrophysiologic testing revealed generalized loss of cone function, with preservation of rod function.19 Furthermore, mutations of cGMP phosphodiesterase 6alpha′-subunit gene leads to progressive cone photoreceptors degeneration in zebrafish, suggesting that PDE6alpha′ activity is important for the survival of cones.20
BIOSYNTHESIS AND TURNOVER OF ROD OUTER SEGMENT MEMBRANES
All of the components of cone and rod outer segments are constantly being renewed by molecules synthesized in the inner segment. The reason for renewal is not known, but it seems reasonable for the cell to have a mechanism to replace old and dysfunctional molecules. In 1976, Young21 described two mechanisms of outer segment renewal: molecular replacement and membrane replacements. In molecular replacement, individual components of the outer segments are replaced molecule for molecule. An example is the soluble proteins of ROS, which diffuse into the outer segment after synthesis in the inner segment. Another example is the exchange of single lipid molecules catalyzed by lipid transfer proteins In membrane replacement, vesicles containing newly synthesized lipids and proteins are transported to the base of the outer segment, where they fuse with the plasma membranes near the connecting cilium.22 The increase in plasma membrane surface area causes the basal membranes of the outer segment to evaginate and fold over (Fig. 12-2). In rod outer segments, these basal unfoldings eventually pinch off to form a free-floating disc.23 In cones, the plasma and disc membranes remain continuous.
Let us follow the synthesis of rhodopsin in the rod photoreceptor cell. The gene that encodes rhodopsin directs the synthesis of a messenger RNA (transcription) specific for rhodopsin. The message is translated on membrane-bound ribosomes, where opsin is synthesized, acetylated, and glycosylated at its N-terminus, palmitoylated at its C-terminus, disulfide bonds established and incorporated into the membrane of the endoplasmic reticulum. This membrane buds and fuses with the Golgi cisternae where the two sugar residues are modified. Finally, vesicles containing newly synthesized rhodopsin are released and make their way to the base of the outer segment. This transport process to the base of the outer segments is an active process that is facilitated by several members of a small GTP-binding protein family known as Rab proteins.24
As new discs are added at the base of the outer segment, membranes at the apical tip are shed and phagocytized by the RPE. It takes about 10 days in mammals for a newly incorporated disc at the base to reach the tip of a ROS. Integral proteins such as rhodopsin, once incorporated into a disc, remain with that disc until it is shed.
Lipids are renewed by both membrane and molecular replacement. The vesicles that contain newly synthesized opsin also contain lipid. When fused with the plasma membrane, these lipids become part of the basal unfoldings that eventually give rise to the discs. However, the retina has soluble lipid transfer proteins that affect a one-for-one exchange of lipids between intracellular membranes.21 Also, studies have shown that lipid incorporation into ROS can proceed in the absence of protein synthesis.25 Thus, both membrane and molecular replacement are important in the renewal of outer segment lipids.
Forty years ago, it was discovered that rods and cones shed their tips at precise times of the day.26 Rods shed in the morning at about the time of subjective sunrise, and cones shed in the evening about the time of subjective sunset. In mammals, these are circadian events whose periodicities are set, but not driven, by light.27 In amphibians, the rod-shedding event can be driven by light.28 Invertebrates also exhibit a similar phenomenon in which light provokes the disruption of their microvilli, followed by a rapid reorganization of their characteristic structure.29 The balance between renewal and shedding maintains a functional outer segment of uniform length throughout the lifetime of the cell. Anything that disrupts this delicate balance can lead to functional anomaly and may cause death of the photoreceptor cell. The best example of this is Royal College of Surgeons (RCS) rats, whose photoreceptors renew properly but whose RPE cannot phagocytize shed ROS tips.30 As a result, RCS photoreceptors degenerate within 2 months after birth.
PHOTOCHEMISTRY OF VISUAL PIGMENTS
PHOTOBLEACHING OF RHODOPSIN
The 11-cis-retinal chromophore of rhodopsin is oriented perpendicular to the fatty acid chains in the lipid bilayer of the ROS discs, a position that enhances photon capture. A single photon can isomerize the cis double bond, producing all-trans-retinal. The conversion of 11-cis-retinal to all-trans-retinal is the only known direct action of light in the photoreceptors and leads to dramatic changes in the three dimensional structure of retinal, which ultimately provokes conformational changes in rhodopsin. These conformational changes have been captured at low temperatures by several investigators who have studied their spectroscopic and kinetic properties. The first stable intermediate at physiologic temperatures is metarhodopsin II, and its formation from metarhodopsin I within milliseconds of photon capture leads to visual excitation. Each of these intermediates is a different conformational state of photoactivated rhodopsin (opsin) that results from the cis to trans isomerization of retinal.
THE VISUAL CYCLE
All-trans-retinal (ATR) produced by the photoactivation of rhodopsin must be isomerized enzymatically to 11-cis-retinal, the substrate for regeneration of rhodopsin. The isomerase enzymes are located in the RPE31; no isomerase activity has been found in the retina. Therefore, the transport of all-trans-retinal to and from the RPE is an important part of the visual cycle, which is depicted in Figure 12-4. ATR released within the photoreceptor discs from photolyzed rhodopsin is first transferred to the cytoplasm by the action of a specialized flippase enzyme ATP-binding cassette transporter (ABCA4) whereby it is rapidly reduced to all-trans-retinol (ATol) by the enzyme retinol dehydrogenase. The cofactor nicotinamide-adenine dinucleotide phosphate in its reduced form (NADPH) is generated by glucose-6-phosphate dehydrogenase, an enzyme of the hexose monophosphate shunt. In low concentrations, ATol can disrupt membranes. Therefore, it must be removed rapidly from the outer segments to the RPE to prevent damage to the discs. The mechanism of transport of ATol to the RPE is not well understood. The interphotoreceptor matrix contains high levels of interphotoreceptor retinoid binding protein (IRPB), which is considered responsible for moving retinoids between the ROS and the RPE.32 However, other evidence has shown that ATol can move between the two cells by simple diffusion.33 Both mechanisms most likely are used. In the RPE, ATol is esterified by the action of the enzyme lecithin-retinol acyltransferase (LRAT) to retinyl ester that is in turn converted to 11-cis retinol by the isomerohydrolase enzyme RPE65.34 Finally, the 11-cis retinol is oxidized to 11-cis retinal by a NAD-dependent RDH.35 11-cis retinal is then delivered to the rod photoreceptors to regenerate a functional pigment. Therefore, aside from the conversion of ATR to ATol, the retinoid cycle for rods is mostly carried out in the RPE.36
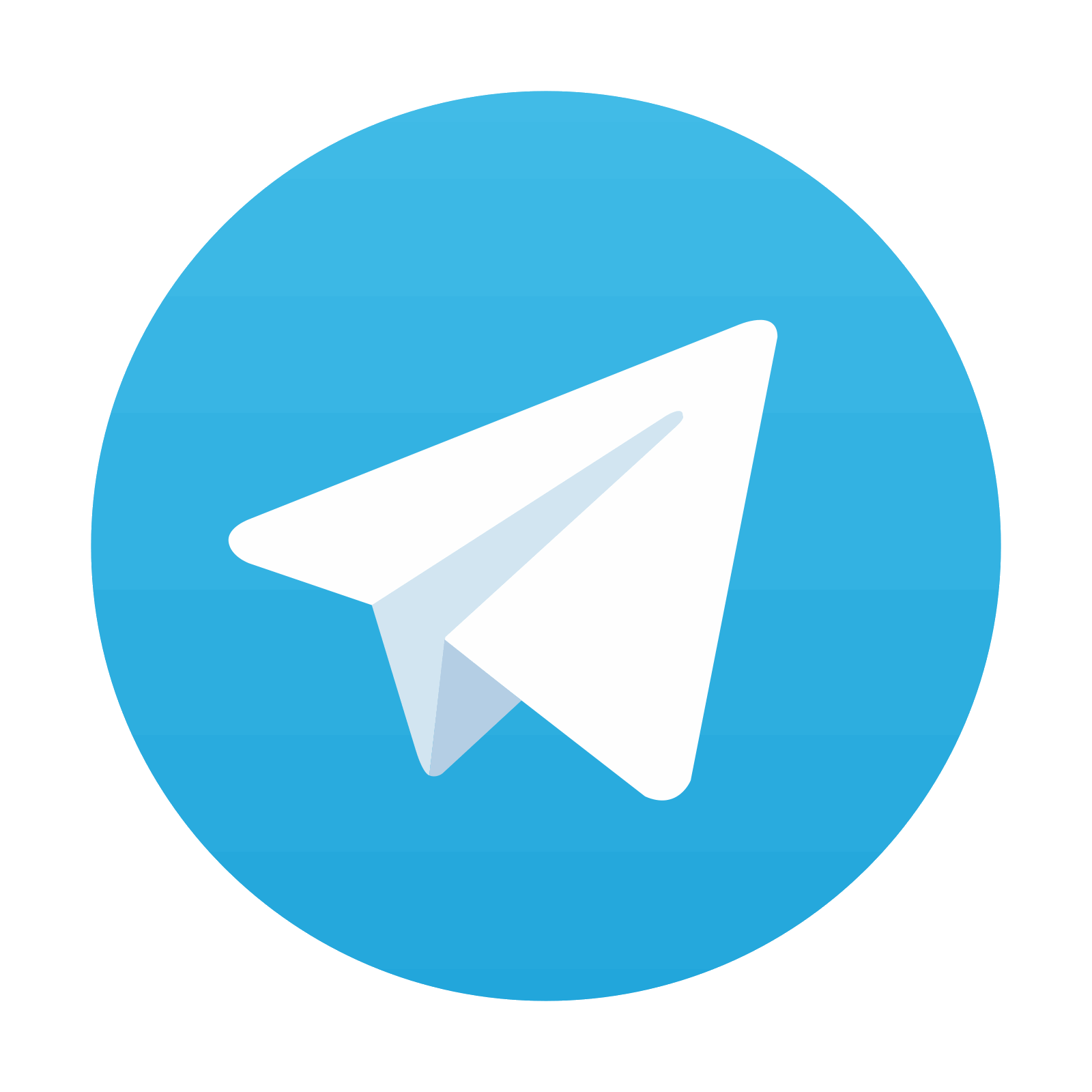
Stay updated, free articles. Join our Telegram channel
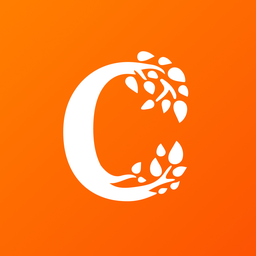
Full access? Get Clinical Tree
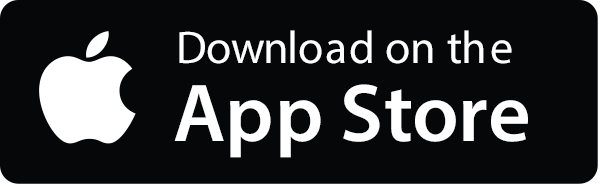
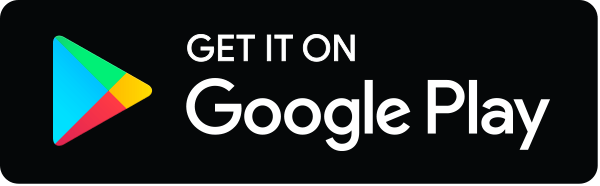