Magnetic Resonance Imaging for the Ophthalmologist
Kiran S. Talekar
Adam E. Flanders
The superior contrast resolution and multiplanar capabilities of magnetic resonance imaging (MRI) make it uniquely qualified for the assessment of the visual pathways. The predecessor to MRI, computed tomography (CT), also produces high-resolution digital images of the brain and orbital structures. However, because CT is based on the same physical principles of the X-ray, it accentuates bony anatomy at the expense of the soft-tissue detail. For example, at locations where soft-tissue components of the orbital contents are in close approximation to bony elements (e.g., the orbital apex, optic foramen), the details of the soft-tissue structures are lost.
Mobile hydrogen protons generate images in MRI, and these hydrogen protons are far more abundant in the soft-tissue structures than in bone. Therefore, the soft tissues are accentuated in MRI at the expense of the bony anatomy. Thus, MRI is well suited for the detection of subtle abnormalities affecting the optic pathways. Disease processes that produce subtle pathologic changes in the optic pathways, such as optic neuritis, are easily identified with an MRI.
MRI uses a strong static magnetic field and radio waves to generate images; it has no known potential harmful effects in biologic tissues. Compared to CT, MRI does not use ionizing radiation; therefore, there is no potential risk of presenile cataracts due to radiation exposure for imaging the orbit. MRI does impose several safety limitations, which preclude imaging of patients with certain implanted ferrous instrumentation, such as cardiac pacemakers, neurostimulators, and older model cerebral aneurysm clips. In addition, approximately 10% of patients will experience an episode of claustrophobia during an MRI that may prevent completion of the entire examination. Sedation may be necessary to complete the examination.
High-field MRI units (1.5 Tesla or greater) using a standard head (brain) coil can produce images of the optic pathways with exceptional detail. No special equipment is required. Although low-field strength open MRI units
have an advantage in imaging claustrophobic patients, their capabilities in resolving small structures (e.g., optic nerves and cranial nerves) is limited because of their low inherent magnetic field strength, longer imaging times, and lower spatial and contrast resolution. Therefore, use of these devices for ophthalmologic imaging should be reserved only as a last resort in preference to a high-field strength unit.
have an advantage in imaging claustrophobic patients, their capabilities in resolving small structures (e.g., optic nerves and cranial nerves) is limited because of their low inherent magnetic field strength, longer imaging times, and lower spatial and contrast resolution. Therefore, use of these devices for ophthalmologic imaging should be reserved only as a last resort in preference to a high-field strength unit.
FUNDAMENTALS OF MRI
MRI is based on the principles of nuclear magnetic resonance. In brief, the images generated with MRI are created by exploiting the principle that the mobile protons in biologic tissues (primarily water and fat) align themselves and resonate along the direction of a strong static magnetic field at a known frequency (Larmor frequency). In a clinical MRI unit, the static magnetic field may vary between 0.3 and 3.0 Tesla (3,000 to 30,000 gauss—or up to 100,000 times the earth’s own magnetic field). During the MR examination, the resonating protons are exposed to a burst of radiofrequency energy that briefly excites them to a higher energy state. After excitation, the protons spontaneously undergo a process of relaxation and release weak radiofrequency energy, which is detected by an antenna (coil) inside the bore of the MRI unit. Through a series of sophisticated mathematical computations, the radio frequency map emitted by the excited tissue is converted into a spatial signal map that appears as an image.
TERMINOLOGY AND MRI PULSE SEQUENCES
In the MRI unit, different tissues and disease processes exhibit tissue-specific relaxation properties that allow one tissue to be distinguished from another. These fundamental relaxation properties are expressed as a rate or units of time and are known as T1 and T2 (Fig. 3-1). Tissues can be described by their T1 and T2 relaxation rates, proton density, and rate of movement (diffusion or blood flow). MRI pulse sequences have evolved from the most basic saturation recovery sequences to the ultrafast Echoplanar imaging sequences. A variety of pulse sequences exist, which are designed to make use of tissue-specific characteristics that improve the conspicuity of particular tissue relative to the background tissues. Similar pulse sequences may have different acronyms depending on the vendor and are therefore confusing. Although a detailed discussion of these pulse sequences is beyond the scope of this chapter, a list of the commonly used sequences is tabulated below. The basic pulse sequences take advantage of differences in the T1 or T2 relaxation properties, otherwise known as T1-weighted or T2-weighted pulse sequences. The term “weighted” is used because while the T1 or T2 relaxation characteristics provide the majority of the tissue information; there are other tissue-specific parameters that provide minor but observable contributions to the “look” of the images. It is useful to learn how to recognize these basic image types. It is also important to realize that as MRI signal and enhancement characteristics are not entirely disease specific, MRI interpretation is largely dependent on the location and morphology of the abnormality in conjunction with the clinical history.
T1-weighted Imaging
It is based on T1 relaxation time of the tissues. In the orbit it gives a good general anatomic outline with the normal retrobulbar fat imparting excellent contrast. It also serves as a baseline to study contrast enhancement characteristics. The T1 signal ranges from very hyperintense for fat to very hypointense for cerebrospinal fluid (CSF). Certain lesions containing fat (dermoid
and lipoma), subacute blood (Figs. 3-2A and 3-3B and D), and melanin are characteristically hyperintense on T1. Fat-suppression techniques help distinguish fat and fatcontaining lesions from other T1 hyperintense lesions. White matter is more hyperintense than gray matter due to abundance of myelin. Therefore, disease processes severely affecting myelin, like progressive multifocal leukoencephalopathy (PML), which targets oligodendrocytes (Fig. 3-4C), are characterized by a very hypointense T1 signal.
and lipoma), subacute blood (Figs. 3-2A and 3-3B and D), and melanin are characteristically hyperintense on T1. Fat-suppression techniques help distinguish fat and fatcontaining lesions from other T1 hyperintense lesions. White matter is more hyperintense than gray matter due to abundance of myelin. Therefore, disease processes severely affecting myelin, like progressive multifocal leukoencephalopathy (PML), which targets oligodendrocytes (Fig. 3-4C), are characterized by a very hypointense T1 signal.
Gadolinium-enhanced T1
This is vital in characterizing lesions. With the possible exception of imaging for optic atrophy, use of contrast material is mandatory when imaging the optic pathways. This sequence is acquired with fat suppression technique while imaging the orbit, as normal fat may obscure pathologic enhancement due to its inherent T1 hyperintensity. The MR contrast agent contains heavy metal gadolinium. Intravenous contrast material is used to improve the visibility of an abnormality (e.g., optic nerve sheath meningioma; Fig. 3-5B and D), to characterize the activity of a pathologic process [e.g., multiple sclerosis (MS); Fig. 3-6B], or to make pathology visible (e.g., optic neuritis; Fig. 3-7D and E, and other cranial neuropathies; Fig. 3-8B). Meningeal pathologies may only be seen on postcontrast sequences (Figs. 3-9B-D and 3-10). The mechanism for contrast enhancement in MRI is similar to the process on CT; it augments areas of increased vascularity or vascular permeability (e.g., damaged blood-brain barrier), which are nonspecific markers for a disease process. Beware of normally enhancing structures, including the pituitary gland (Figs. 3-1K, 3-11B, and 3-12C), pituitary stalk (Fig. 3-7E), veins (like superior ophthalmic veins), and adjacent nasal mucosa (Fig. 3-13B). Symmetric enhancement is to be expected in the extraocular muscles (Fig. 3-1I and J) and lacrimal glands. Additionally, susceptibility artifacts from air in the nasal cavity and paranasal sinuses and from dental works may mimic enhancement, especially on 3-Tesla MRI.
T2
It is based on T2 weighting of the tissues (Fig. 3-1B and H). This sequence is very sensitive for detecting pathology but is not entirely specific. CSF and most pathologies (due to higher water content compared to normal tissues) are hyperintense: For example, demyelination (Figs. 3-4B and 3-6D), tumors (Fig. 3-5C), infection (Fig. 3-14B), and strokes (Fig. 3-15C). Cavernous hemangiomas are characteristically extremely bright on T2 while tumors like lymphoma and meningioma may be less hyperintense secondary to high cellularity. Heavily T2W high-resolution sequences can be obtained in patients with cranial neuropathies to study the cisternal segments of the nerves (Fig. 3-1L-N).
Short T1 Inversion Recovery and T2 Fat Saturated Sequence
In these techniques, hyperintense signal from normal fat is suppressed on an essentially T2-weighted image (Fig. 3-1D-G), making lesions and edema evident, which is vital in detecting subtle T2 hyperintensity in the optic nerve (3-7A-C and 3-16B). In general, the ability to perceive an abnormality is markedly improved with the use of a fat-suppression technique. Additionally it is vital in detecting calvarial marrow abnormalities including osteomyelitis and tumors.
Fluid-attenuated Inversion Recovery
The fluid-attenuated inversion recovery (FLAIR) is also essentially T2WI but with active suppression of signal arising from bulk water (cerebrospinal fluid) more than from bound water (interstitial edema or demyelination). This is the most sensitive technique to
demonstrate periventricular white matter disease (e.g., demyelinating lesions; Fig. 3-6A and C) and subarachnoid disease processes (e.g., subarachnoid hemorrhage and leptomeningeal inflammation or carcinomatosis). Other examples included are infarct (Fig. 3-15A), tumor (Figs. 3-13A), and inflammatory neuritis (Fig. 3-8A).
demonstrate periventricular white matter disease (e.g., demyelinating lesions; Fig. 3-6A and C) and subarachnoid disease processes (e.g., subarachnoid hemorrhage and leptomeningeal inflammation or carcinomatosis). Other examples included are infarct (Fig. 3-15A), tumor (Figs. 3-13A), and inflammatory neuritis (Fig. 3-8A).
T2W Gradient Echo
This is obtained with the specific purpose of detecting susceptibility artifact primarily from blood products (Fig. 3-17B). However, other material including calcification, gas, and metallic structures like surgical clips and aneurysm coils cause susceptibility artifact.
Proton Density Weighted
Proton density weighted (PDW) image contrast is purely based on differences in the number of protons between tissues. It is no longer a common sequence. Some argue that it is helpful in detecting demyelinating lesions. Figure 3-18A shows an unusual application of this sequence demonstrating abnormal signal in an aneurysm.
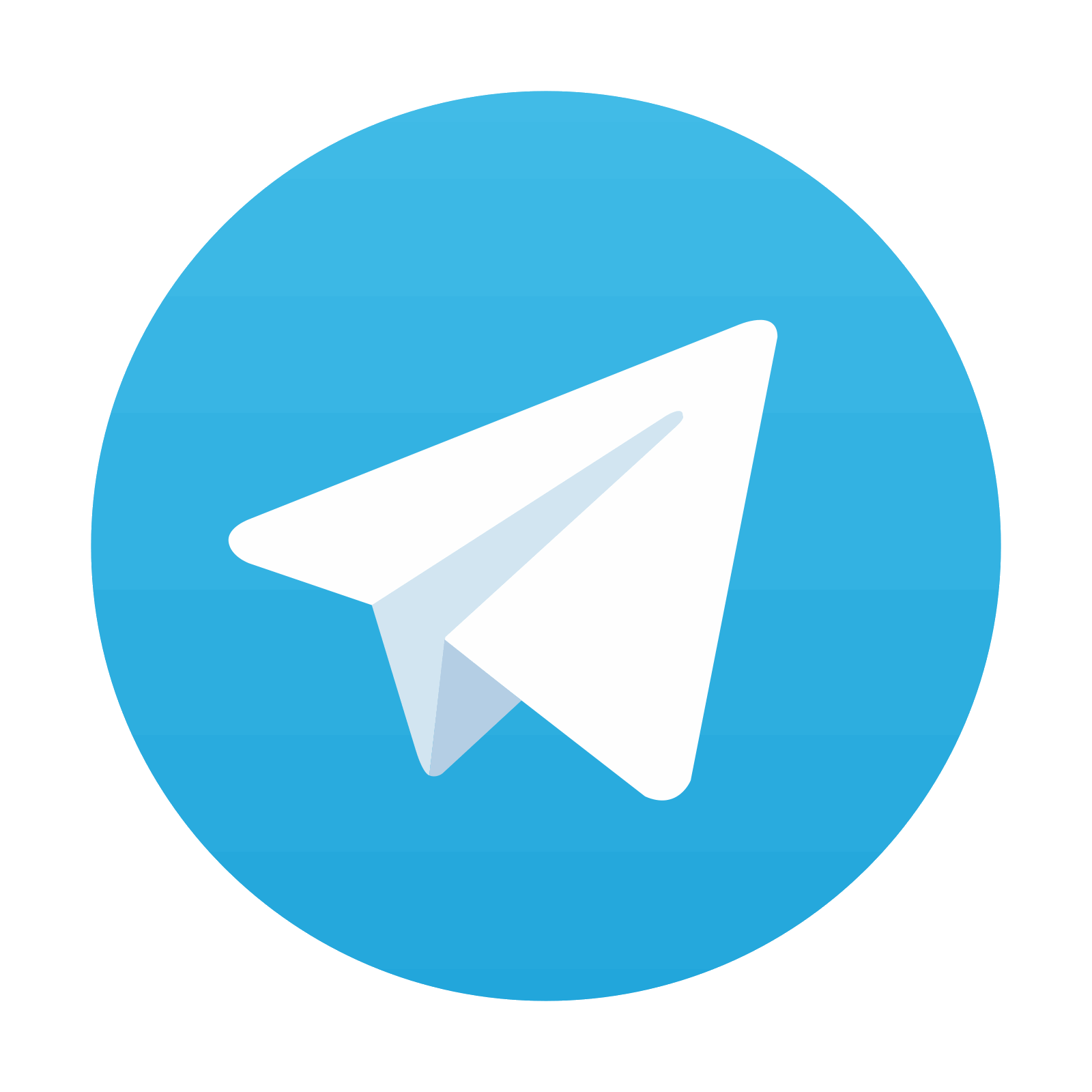
Stay updated, free articles. Join our Telegram channel
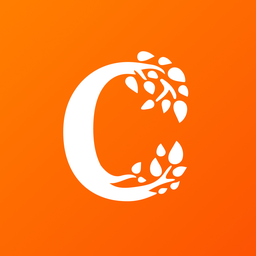
Full access? Get Clinical Tree
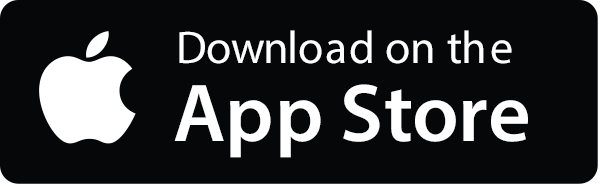
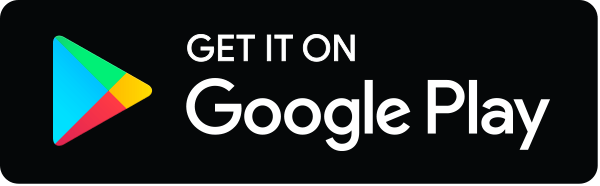
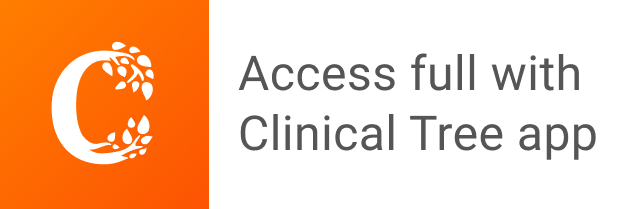