3
Laryngeal Physiology
A thorough understanding of laryngeal physiology provides the foundation for the interpretation of laryngeal imaging in the evaluation of patients with laryngeal disorders. Once the clinician understands the interactions between the laryngeal structures and the pulmonary system that result in voice, it becomes clear that almost every case of laryngeal pathology interrupts the normal laryngeal physiology in a predictable way. Throughout the chapters in this book, authors will refer back to a discussion of the essential elements of normal laryngeal physiology to explain abnormalities of vibration or the impact of various pathologies on voice. This chapter describes the essential elements of normal laryngeal functioning and the unique histology of the larynx that allows the generation of the sound we all know as the human voice.
The human larynx is a highly specialized organ that provides many unique functions. Its primary function is as a gateway to the upper respiratory tract, and it plays an integral role in facilitating breathing, swallowing, and phonation. Laryngeal function and physiology relies on a sophisticated network of neural signaling, neuromuscular control, respiratory regulation, and vocal fold vibratory mechanics. A complete understanding of these complex interactions is still a distant goal of researchers, but much has been accomplished.
Developmental Adaptations of the Larynx
The most primitive larynx (Polypterus, lung-fish) serves as a sphincter to protect the lower airways from food or other foreign bodies.1 Additionally, discrete muscular fibers provide active dilation of the glottic opening. With the need for additional ventilation in a terrestrial environment, active glottal dilation is enhanced by the acquisition of lateral cartilages that serve as a site for insertion of the dilator musculature. A cartilaginous ring arising between the glottis and the trachea on which these lateral cartilages sit adds further structural support and prevents collapse of this part of the respiratory system.2 This phylogenetic development can be found in certain types of vertebrates such as reptiles. Phonation is a late adaptation, wherein the larynx functions like a flutter valve. This phenomenon is found only in mammals that have the appropriate respiratory machinery to power the vocal folds, including thoracoabdominal musculature. Among mammals, humans have developed the ability to create intricate sounds and spoken language using the glottis as a vibratory sound source to power the resonators of the pharynx, oral cavity, and nasopharynx.
The separation of the upper aerodigestive tract into channels for breathing and deglutition is a basic finding among all mammals. Furthermore, the larynx is in a relatively high position in close proximity to the posterior nasal cavity. This nasolaryngeal relationship provides a continuous airway from the nose to the lungs thus decreasing the risk of pulmonary compromise from swallowed food and permitting some species to smell approaching predators while grazing.2 The human infant demonstrates a similar relationship with the close location of the epiglottis to the posterior soft palate. A common airway is formed that protects the newborn from aspiration. This relationship is lost after 6 months of age in humans, as the larynx descends in the neck.3 At the base of the tongue, the epiglottis is uniquely shaped to direct food laterally to the pyriform sinuses and away from the midline glottis. A fold of tissue running between the epiglottis and the lateral cartilages (also termed the arytenoid cartilages), or the aryepiglottic folds, acts as a further barrier, directing food toward the lateral pharyngeal walls and away from the laryngeal opening or glottis. Thus, many of the central adaptations of the human larynx serve to facilitate its primary function of airway protection.
Fig. 3.1 Vocal fold histologic section demonstrating structural layers. (From THIEME Atlas of Anatomy, Neck and Internal Organs, © Thieme 2006. Illustration by Markus Voll.)
Vocal Fold Histology
The vocal fold, or vocal cord, is a fold of tissue spanning the distance between the anterior aspect of the arytenoid cartilages and the inner surface of the front of the thyroid cartilage. This fold of tissue has a complex histologic organization that permits it to vibrate when air is passed across the tissue. To understand fully vocal fold vibratory functioning, it is important to describe the histologic complexity of the vocal fold (Fig. 3.1).
The vocal fold consists of five histologic layers: epithelium; superficial, intermediate, and deep layers of the lamina propria; and the thyroarytenoid muscle. The surface epithelium contains stratified squamous cells devoid of mucous glands, unlike the pseudo-stratified ciliated, columnar epithelium found in the remaining respiratory tract.4 This modification allows the vocal fold to maintain shape and undulate freely over the underlying vocalis muscle.
A complex basement membrane zone connects the epithelium of the vocal fold to the superficial lamina propria (SLP).5 The SLP is also known as Reinke’s space and lies immediately deep to the surface epithelium. The basement membrane zone contains a delicate network of proteins and collagen fibers that allow the epithelium to adhere to the gelatinous SLP.6 The SLP layer contains loose fibrous tissue and a network of hyaluronic acid, mucopolysac-charides, decorin, and other extracellular matrix (ECM) components. The SLP contains very few fibroblasts and is primarily devoid of both elastic and collagen fibers. It provides little resistance to vibration and is very flexible, characteristics that are required for proper phonation to occur. Any change in the composition of the ECM can lead to a loss of vibratory function. In particular, alterations in levels of hyaluronan and fibronectin have been found in models of pathologic vocal fold lesions.7 This has created significant interest in the development of novel therapeutic interventions for vocal fold scarring, including use of autologous fibroblasts and hyaluronan hydrogel.7,8
The intermediate layer of the lamina propria is similar to the SLP but contains higher amounts of collagen and mature elastin fibers arranged longitudinally. It is a highly hydrated structure, rich in fibrous and interstitial proteins as well as glycosaminoglycans and proteoglycans. The intermediate layer of the lamina propria also contains large quantities of hyaluronic acid that may act as a shock absorber.
The deep layer of the lamina propria is dense and contains the highest concentration of fibroblasts and collagen fibers. The properties of collagen limit its ability to stretch; therefore, the presence of this layer prohibits overextension of the vocal fold. The deep layer of the lamina propria is 1 to 2 mm thick. Together with the intermediate layer of the lamina propria, the deep layer forms the vocal ligament. The vocal ligament also forms the uppermost portion of the conus elasticus.
The deepest layer of the vocal fold consists of intrinsic laryngeal muscle, namely the thyroarytenoid or vocalis muscle.
A few histologic variations of clinical and physiologic importance exist at the anterior and posterior borders of the membranous vocal fold. Anteriorly, the intermediate layer of the lamina propria thickens to form an area called the anterior macula flava. This structure connects to the anterior commissure tendon, also known as Broyle’s tendon, which provides a transition zone from the inner perichondrium of the thyroid cartilage.9,10 Similarly, posteriorly, the intermediate layer of the lamina propria of the vocal fold thickens again to form the posterior macula flava, which transitions into the arytenoid cartilage. These modifications are thought to facilitate a transition from the membranous vocal fold to the stiffer thyroid and arytenoid cartilage. In addition, they may serve to protect and cushion the vocal fold from damage during phonatory vibration and may contain stem cells with which to repopulate the vocal fold tissues.9,10
The biomechanics of fold vibration is intimately associated with the fold’s layered structure. The five histologic layers of the vocal fold function as three different mechanical groups (Fig. 3.2). The epithelium and SLP make up the cover of the vocal fold; the intermediate and deep layers of the lamina propria make up the vocal ligament or the transition; and the thyroarytenoid muscle makes up the body of the vocal fold. The relationship between these three layers and the gradient of increasing stiffness provides the mechanics for the complex mucosal wave. Vibration of the vocal fold cover (epithelium and SLP) results in the rhythmic opening and closing of the folds, which is critical to the creation of the intermittent pulses of air that we perceive as sound. Stiffness in the cover will result in impairment of vibration and hoarseness. The special components (cells, proteins, matrix scaffolding) and organization of the lamina propria layers (superficial, intermediate, and deep) are all crucial in vocal fold vibration. Any changes to any of these components results in decreased pliability, thus altering vocal fold vibration. It is important for surgeons to keep in mind that unintentional violation of the transition zone during surgical excision of superficial lesions of the vocal fold can lead to scarring of the epithelium to the deeper tissue layers and stiffness. On the other hand, decorin, found in the superficial layer of the lamina propria, reduces fibrosis and scarring after injury. Therefore, surgery and inflammation limited to the SLP is less likely to result in scar formation. The body (vocal ligament and vocalis muscle) of the vocal fold affects vocal fold tension and regulates the resistance to airflow.
Fig. 3.2 Body-cover theory of vibration.
Laryngeal Respiratory Physiology
The larynx has an integral role in respiration, although its primary role in this regard remains a protective one. By closing during deglutition, it guards the lower airways from ingested or inhaled materials. The importance of the larynx as a valve that regulates airflow, both in inspiration and expiration, has been clearly established.11 A variety of laryngeal receptors under central nervous system control also exists to regulate both protective and pathologic glottal reflexes. The glottal inlet generally opens with inspiration and closes reflexively during swallowing and in response to chemical or tactile stimulation.
The larynx regulates resistance to airflow in and out of the lungs and is protected from inspiratory collapse by the rigid cricoid cartilage, which forms a complete ring around the laryngeal airway.11 The vocal folds form a laryngeal valve mechanism that regulates the transglottic pressure difference during both respiration and phonation.12 Laryngeal and upper tracheal airflow is predominately turbulent as a result of the significant reduction in cross-sectional area at the level of the glottis.13
In the resting breathing state, contraction of the posterior cricoarytenoid (PCA) muscles results in abduction of the vocal folds during inspiration, providing a patent laryngeal airway.14 The synchronous action of the cricothyroid muscles may contribute slightly to this dilatory function.15 The vocal fold movement during inspiration is coordinated such that abduction occurs just before diaphragmatic contraction. This provides a mechanical advantage by preventing airflow from occurring against a closed glottis.11 The passive relaxation of the PCA muscles during expiration allows for adduction of the vocal folds, with the other adductory muscles such as the thyroarytenoid muscle providing some support and a small amount of positive end expiratory pressure slowing the rate of exhalation and preventing collapse of the distal bronchioles.
During hyperpneic and hyperthermic states of breathing, and as a result of underlying hypercapnia, the vocal folds abduct more widely during inspiration. This phenomenon continues into the expiratory phase and significantly reduces laryngeal resistance in addition to decreasing both the rate and duration of expiration.16
The larynx has an abundant network of afferent sensory innervation that facilitates feedback control of its protective mechanisms. The afferent fibers run primarily through the internal branch of the superior laryngeal nerve, although a few fibers are also transmitted via the recurrent laryngeal nerve.17 Stimulation of sensory afferent receptors in the larynx can lead to sustained reflex adduction of the vocal folds. The underlying mechanism of a cough can be a result of similar laryngeal stimulation or coughing can be voluntary if mucus or foreign matter needs to be ejected from the lower airways. Coughs are initiated with a preparatory inspiratory phase where an increased inspiratory effort leads to exaggerated abduction of the vocal folds. This is followed by a compressive phase as the glottis tightly closes and intrapulmonary and subglottic pressure rises in response to expiratory muscle contraction. Finally, the cough is terminated with an abrupt opening of the vocal folds, allowing air to rush through at high velocities.18,19
The false vocal folds are also important during coughing, as they function as exit valves to prevent the egress of air from the trachea until sufficient subglottic pressure has been generated. In a closed position, they seal together tightly when pressure from the lower airway increases. The phenomenon of adducted false vocal folds is due to their unique shape and configuration and occurs independently of muscle tone.2 Thus, expectorative functions of the larynx remain unimpaired even in bilateral laryngeal paralysis because passive closure of the false vocal folds is sufficient for effective cough production.
Several types of laryngeal receptors exist that control a variety of sensory and maladaptive reflexes. Mechanoreceptors include “pressure,” “cold,” and “drive” receptors.20 Airflow receptors are activated by cooling of the laryngeal mucosa during inspiration. Other “drive” receptors respond to laryngeal movements or motion during breathing.21 Laryngeal chemore-ceptors are activated by various chemical and noxious stimuli, including liquids such as water. Intralaryngeal water can trigger an apnea reflex and is thought to play a role in the pathogenesis of sudden infant death syndrome.22 Protective reflex glottal closure is usually replicated by direct electrical stimulation of the superior laryngeal nerve, but it can also be triggered by a variety of sensory stimuli. Afferent innervation of the laryngeal adductor reflex occurs via the internal branch of the superior laryngeal nerve and motor action from the recurrent laryngeal nerve branch of the vagus nerve. One example of a pathologic exaggeration of this reflex is laryngospasm. Laryngospasm leads to forceful and prolonged glottal closure that usually lasts long after the offending stimulus has ceased. Typical irritants that can induce laryngospasm include instrumental laryngeal manipulation during general anesthesia, airway foreign bodies, and laryngopharyngeal reflux.19
Laryngeal stimulation can also lead to the activation of a multitude of cardiovascular reflexes. These changes are usually found during intubation or extubation under general anesthesia or when the larynx is exposed to inhaled irritants.23 Systemic hypertension and profound bradycardia have most commonly been described in previous studies.24,25 The receptors for these reflexes have been characterized as polymodal and nociceptive although little is known about their morphology.
Laryngeal Contribution to Swallowing
The mechanism of swallowing is controlled by the central nervous system and consists of both supratentorial and brain-stem components. The supratentorial component is found in the frontal cortex anterior to the sensorimotor cortex.26 The brain-stem components are found in the dorsal aspect of the nucleus tractus solitarius in addition to the ventral aspect of the nucleus ambiguous.27 Cortical and subcortical areas of the brain are integral for voluntary initiation of swallowing, whereas the brain stem is responsible for the involuntary stages of swallowing.28
Swallowing can be divided into three stages: oral, pharyngeal, and esophageal. The oral stage is controlled by voluntary neuromuscular function, whereas the pharyngeal and esophageal stages are involuntary. Intact laryngeal function is most crucial to the pharyngeal stage. During the pharyngeal stage, the larynx serves to primarily protect the airway while inspiration is necessarily inhibited.
At the initiation of the pharyngeal phase of swallowing, the larynx and the hyoid elevate and are pushed forward toward the tongue base. This movement enlarges the pharynx and creates a vacuum or negative pressure in the hypopharynx and larynx, thus allowing the food bolus to be pushed downward. This movement also contributes to the relaxation of the cricopharyngeus muscle. Next, and most importantly, the true and false vocal folds adduct, with closure beginning at the level of the true vocal folds and progressing up to the false vocal folds and then to the aryepiglottic folds. True vocal fold closure is the primary laryngopharyngeal protective mechanism that prevents aspiration during the pharyngeal swallowing stage. The epiglottis then descends over the superior portion of the larynx and protects the airway by diverting the ingested material toward and into the pyriform sinuses. If the ingested material is of a liquid consistency, then the epiglottis acts to slow the liquid movement through the pharynx, giving the vocal folds additional time to adduct and the larynx time to elevate.
The intricate nature of laryngeal movements and reflexes during the pharyngeal swallow stage can lead to significant aspiration and dysphagia when laryngopharyngeal sensation is compromised. This subject has been studied extensively in recent times, and new technology has emerged that allows for sensory testing during endoscopic examination of swallowing.29 These studies have confirmed the importance of sensory feedback control during pharyngeal swallowing, although the mechanism has yet to be delineated completely.30,31
Laryngeal Motion and Vibratory Physiology
Motor innervation to the intrinsic laryngeal musculature originates in the medullary nucleus ambiguus. The action of the intrinsic laryngeal muscles primarily determines vocal fold shape and movement. These muscles dictate the extent of abduction, adduction, length, mass, stiffness, and tension of the vocal folds (see Chapter 2, Figs. 2.7, 2.9, and 2.10). These six biomechanical parameters profoundly alter the vibratory characteristics of the vocal folds and thus the nature of the sound produced during vibration or phonation.
The chief adductory muscles include the thyroarytenoid, lateral cricoarytenoid (LCA), and interarytenoid. The LCA muscle originates from the lateral aspect of the cricoid cartilage and inserts onto the muscular process of the arytenoid. The thyroarytenoid muscle originates from the inner thyroid cartilage and inserts onto the muscular process of the arytenoid. The thyroarytenoid forms the body of the vocal folds and it also shortens, lowers, and thickens the vocal folds, in addition to altering the intrinsic stiffness of the folds during contraction. In this way, contraction of the thyroarytenoid muscle increases the resistance to airflow through the glottis. The LCA muscle is the stronger adductor, and its activity includes elongating and thinning the vocal fold, but it primarily functions to close the posterior portion of the folds.32 Males require the posterior closure to generate enough subglottal pressure to set the folds into vibration. Conversely, women may not demonstrate complete posterior closure and do not need the extra subglottal pressure to phonate due to their less massive folds. The PCA muscle is the only abductory muscle. It originates at the posterior surface of the cricoid cartilage and inserts onto the muscular process of the arytenoid. Its action is accomplished by pulling the muscular process both posteriorly and inferiorly.
The PCA muscle also elevates, elongates, and thins the vocal fold and provides stabilization of the arytenoids allowing the other intrinsic muscles to function more efficiently. The cricothyroid muscle originates from the cricoid cartilage and has dual insertions into both anterior and posterior aspects of the thyroid cartilage lamina.33 Contraction of the cricothyroid muscles increases vocal fold tension and length by increasing the distance between the posterior cricoid cartilage and the anterior commissure. This muscle is central to controlling and increasing vocal pitch by its ability to thin and simultaneously stretch the fold.
The normal vibratory-phonatory cycle is regulated by several principles that include adequate respiratory support, appropriate glottal closure, an intact vocal fold cover, and fine-tuned control of vocal fold length and tension.9 The vibrating vocal folds convert the airflow and pressure energy generated by the lungs, the diaphragm, and the thoracoabdominal musculature into acoustic power. Many factors affect the production of sound at the glottal level, and these include subglottal pressure, glottal impedance, volume velocity of glottal airflow, and supraglottal pressure.10
Voice is achieved by a complex repeating cycle in which glottal opening and closing modulates the transglottic air-stream at anywhere from 50 to 1000 cycles per second. Despite many years of mathematical and tissue modeling studies on the nature of fold vibration, the essential factors permitting sustained vibration of the vocal folds is not yet understood. However, the series of events characterizing a vibratory cycle have been described. Each cycle begins with the subglottic pressure pushing against the undersurface of the closed vocal folds. The pressure from the lungs eventually overcomes the medial closing forces holding the folds together, also termed the phonatory threshold pressure, and pushes the vocal folds apart. The inferior-most part of the folds opens first, and the tissue is progressively compressed as an “air bubble” rises to the superior surface of the folds. This compression from inferior to superior is called a traveling wave. When the “bubble” or wave reaches the superior portion of the folds, they start to unzip, and pressurized air begins to escape from the folds as a jet. This jet can attain velocities of 50 or more meters per second. The vocal folds open first anteriorly.
At the superior portion of the folds, the tissue wave propagates out laterally. However, during this lateral wave propagation, the inferior-most portion of the folds now begins to move medially as a result of many factors, including a decrease in subglottic pressure, the recoiling force generated by the elasticity of the vocal folds, and a vacuum-like negative pressure phenomenon created by the Bernoulli effect.9 The vocal folds contact again inferiorly, and this closure then progresses superiorly and anteriorly until the vocal folds close completely, leading to repetition of the cycle (Figs. 3.3 and 3.4). Studies of the medial surface of the vocal folds have confirmed significantly greater medial-lateral and vertical displacements than anterior-posterior displacement.34 Sound is produced during fold oscillation by the inertial interaction of the pulsating jet against the air columns above and below the folds creating an air pressure wave that then interacts with the resonators of the vocal tract before exiting the lips. It is known that the maximum degree of air pressure modulation occurs at the moment of closure of the inferior vocal fold margin. In addition, the sound produced by the folds is complex in nature having both a fundamental frequency and associated harmonics decreasing at 12 dB per octave.
Fig. 3.3 Transglottic airflow and vocal fold vibratory cycle.
The simplest models of phonation are based on the principle that pitch depends on the frequency of vocal fold vibration, which is related to the vocal fold length, whereas loudness is the result of subglottic pressure and the amplitude of vibration of the vocal folds. Increases in subglottic pressure require greater resistance to airflow at the level of the glottis and greater air pressure generated by the lungs. Studies of vocal fold oscillation suggest, however, that the velocity and wavelength of the traveling wave controls the frequency of vibration.35,36 As each traveling wave travels faster, the frequency of vibration increases and so does the pitch of the voice. Similarly, as the folds are thinned, the effective wavelength decreases, and the frequency of vibration increases along with the perceived pitch of the voice. Central neuromotor control of the intrinsic laryngeal muscles affects traveling wave characteristics. In other words, alteration in laryngeal muscle activity affects the phonatory sound quality. Thus, through central neuromuscular control, voice quality is altered.
Fig. 3.4 Vocal folds viewed from above during one vibratory cycle. The anterior aspect of the vocal folds opens first with opening progressing posteriorly. The posterior aspect of the vocal folds closes first with closure progressing anteriorly.
The three main types of vibratory patterns that exist are modal, falsetto, and glottal fry. In the modal register, the vocal folds exhibit a normal vibratory topography as the mucosa vibrates independently of the muscle. In falsetto, glottal closure is incomplete, and only the uppermost free edges of the folds are involved in vibration, creating a high-pitched voice. Glottal fry is characterized by an excessively low-pitched voice with the vocal folds tightly approximated for a longer than normal duration during the vibratory cycle. These modes of phonation can be further characterized by the analysis of the open and closed phases of the vibratory cycle. The opening phase begins once the vocal fold margins are elevated and ends when the opening is maximal. A closing phase follows, which is finalized when the vocal fold margins are adjoining. The relationship between the two phases is based on the vocal pitch and intensity. During loud phonation, the closing phase is longer than the opening phase. The pitch also influences the closing phase, and the higher the pitch, the shorter the closing phase. The amplitude of displacement correlates positively with the intensity of phonation and negatively with the fundamental frequency and is decreased for breathy and pressed phonation modes.
Phonation would be incomplete without the modulation of the glottal output by the resonators in the chest, pharynx, and nasal cavities. These differing vocal tract configurations act as filters that modify glottal phonation to form the sound that is perceived as the human voice. Recent clinical research has led to the description of unique aerodynamic phenomena such as glottal and supraglottal vortical flow that may also significantly contribute to phonation.37 Distortions in these rotational motions have been found in a variety of laryngeal pathologies, including disorders with asymmetric vocal fold tension.38 In addition, the development of an ex vivo larynx model has the potential to facilitate the measurement of glottal variables in a neuromuscularly correct model.39
Laryngeal Physiology and High-Speed Imaging
The advent of high-speed digital videoendoscopy has revolutionized laryngeal imaging. Digital video systems now exist with the ability to capture up to 10,000 high-resolution color images per second.40,41 Stroboscopy is limited in its ability to provide information about individual vibratory cycles. High-speed imaging, on the other hand, has the potential to increase our understanding of vibratory physiology exponentially. The combination of laser technology with high-speed imaging systems has led to accurate measurements of vocal fold length, vibratory amplitude, glottal area, and vertical mucosal wave movements. Investigators are now using these systems to provide detailed visual playback of mucosal wave dynamics and vibratory symmetry.41 The future of phonatory physiology is truly exciting and promising, as clinicians and researchers continue to uncover fascinating details about vocal fold vibration.
References
1. Negus VE. The Comparative Anatomy and Physiology of the Larynx. London, UK: Heinemann; 1949
2. Sasaki CT. Physiology of the larynx. In: English G, ed. Otolaryngology. Hagerstown, MD: Harper and Row; 1984
3. Sasaki CT, Levine PA, Laitman JT, Crelin ES Jr. Postnatal descent of the epiglottis in man: a preliminary report. Arch Otolaryngol 1977;103:169–171
4. Nassar VH, Bridger GP. Topography of the laryngeal mucous glands. Arch Otolaryngol 1971;94:490–498
5. Gray SD. Cellular physiology of the vocal folds. Otolaryngol Clin North Am 2000;33:679–698
6. Gray SD, Pignatari SS, Harding P. Morphologic ultra-structure of anchoring fibers in normal vocal fold basement membrane zone. J Voice 1994;8:48–52
7. Thibeault SL. Advances in our understanding of the Reinke space. Curr Opin Otolaryngol Head Neck Surg 2005;13:148–151
8. Chhetri DK, Head C, Revazova E, Hart S, Bhuta S, Berke GS. Lamina propria replacement therapy with cultured autologous fibroblasts for vocal fold scars. Otolaryngol Head Neck Surg 2004;131:864–870
9. Noordzij JP, Ossoff RH. Anatomy and physiology of the larynx. Otolaryngol Clin North Am 2006;39:1–10
10. Sataloff RT, Heman-Ackah YD, Hawkshaw MJ. Clinical anatomy and physiology of the voice. Otolaryngol Clin North Am 2007;40:909–929, v
11. Bartlett D Jr. Respiratory functions of the larynx. Physiol Rev 1989;69:33–57
12. Proctor DF. Breathing, Speech, and Song. Vienna, Austria: Springer-Verlag; 1980
13. Olson DE, Sudlow MF, Horsfield K, Filley GF. Convective patterns of flow during inspiration. Arch Intern Med 1973;131:51–57
14. Suzuki M, Kirchner JA. The posterior cricoarytenoid as an inspiratory muscle. Ann Otol Rhinol Laryngol 1969;78:849–864
15. Suzuki M, Kirchner JA, Murakami Y. The cricothyroid as a respiratory muscle. Its characteristics in bilateral recurrent laryngeal nerve paralysis. Ann Otol Rhinol Laryngol 1970;79:976–983
16. England SJ, Bartlett D Jr. Changes in respiratory movements of the human vocal cords during hyperpnea. J Appl Physiol 1982;52:780–785
17. Mathew OP, Sant’Ambrogio G, Fisher JT, Sant’Ambrogio FB. Respiratory afferent activity in the superior laryngeal nerves. Respir Physiol 1984;58:41–50
18. Macklem PT. Physiology of the cough. Ann Otol Rhinol Laryngol 1974;83:761–768
19. Nishino T, Tagaito Y, Isono S. Cough and other reflexes on irritation of airway mucosa in man. Pulm Pharmacol 1996;9:285–292
20. Mathew OP, Sant’Ambrogio G, Fisher JT, Sant’Ambrogio FB. Laryngeal pressure receptors. Respir Physiol 1984;57:113–122
21. Sant’Ambrogio G, Mathew OP, Fisher JT, Sant’Ambrogio FB. Laryngeal receptors responding to transmural pressure, airflow and local muscle activity. Respir Physiol 1983;54:317–330
22. Downing SE, Lee JC. Laryngeal chemosensitivity: a possible mechanism for sudden infant death. Pediatrics 1975;55:640–649
23. Nishino T, Kochi T, Ishii M. Differences in respiratory reflex responses from the larynx, trachea, and bronchi in anesthetized female subjects. Anesthesiology 1996;84:70–74
24. Tomori Z, Widdicombe JG. Muscular, bronchomotor and cardiovascular reflexes elicited by mechanical stimulation of the respiratory tract. J Physiol 1969; 200:25–49
25. Prys-Roberts C, Greene LT, Meloche R, Foëx P. Studies of anaesthesia in relation to hypertension. II. Haemodynamic consequences of induction and endotracheal intubation. Br J Anaesth 1971;43: 531–547
26. Jean A, Car A. Inputs to the swallowing medullary neurons from the peripheral afferent fibers and the swallowing cortical area. Brain Res 1979;178: 567–572
27. Jean A. Brainstem organization of the swallowing network. Brain Behav Evol 1984;25:109–116
28. Martin RE, Sessle BJ. The role of the cerebral cortex in swallowing. Dysphagia 1993;8:195–202
29. Aviv JE, Martin JH, Keen MS, Debell M, Blitzer A. Air pulse quantification of supraglottic and pharyngeal sensation: a new technique. Ann Otol Rhinol Laryngol 1993;102:777–780
30. Jafari S, Prince RA, Kim DY, Paydarfar D. Sensory regulation of swallowing and airway protection: a role for the internal superior laryngeal nerve in humans. J Physiol 2003;550(Pt 1):287–304
31. Setzen M, Cohen MA, Perlman PW, et al. The association between laryngopharyngeal sensory deficits, pharyngeal motor function, and the prevalence of aspiration with thin liquids. Otolaryngol Head Neck Surg 2003;128:99–102
32. Nasri S, Sercarz JA, Azizzadeh B, Kreiman J, Berke GS. Measurement of adductory force of individual laryngeal muscles in an in vivo canine model. Laryngoscope 1994;104:1213–1218
33. Hong KH, Ye M, Kim YM, Kevorkian KF, Kreiman J, Berke GS. Functional differences between the two bellies of the cricothyroid muscle. Otolaryngol Head Neck Surg 1998;118:714–722
34. Doellinger M, Berry DA, Berke GS. A quantitative study of the medial surface dynamics of an in vivo canine vocal fold during phonation. Laryngoscope 2005;115:1646–1654
35. Nasri S, Sercarz JA, Berke GS. Noninvasive measurement of traveling wave velocity in the canine larynx. Ann Otol Rhinol Laryngol 1994;103:758–766
36. Sloan SH, Berke GS, Gerratt BR, Kreiman J, Ye M. Determination of vocal fold mucosal wave velocity in an in vivo canine model. Laryngoscope 1993;103: 947–953
37. Khosla S, Muruguppan S, Gutmark E, Scherer R. Vortical flow field during phonation in an excised canine larynx model. Ann Otol Rhinol Laryngol 2007;116: 217–228
38. Khosla S, Murugappan S, Gutmark E. What can vortices tell us about vocal fold vibration and voice production. Curr Opin Otolaryngol Head Neck Surg 2008;16:183–187
39. Berke GS, Neubauer J, Berry DA, Ye M, Chhetri DK. Ex vivo perfused larynx model of phonation: preliminary study. Ann Otol Rhinol Laryngol 2007;116:866–870
40. Mehta DD, Hillman RE. Voice assessment: updates on perceptual, acoustic, aerodynamic, and endoscopic imaging methods. Curr Opin Otolaryngol Head Neck Surg 2008;16:211–215
41. Deliyski DD, Petrushev PP, Bonilha HS, Gerlach TT, Martin-Harris B, Hillman RE. Clinical implementation of laryngeal high-speed videoendoscopy: challenges and evolution. Folia Phoniatr Logop 2008;60:33–44
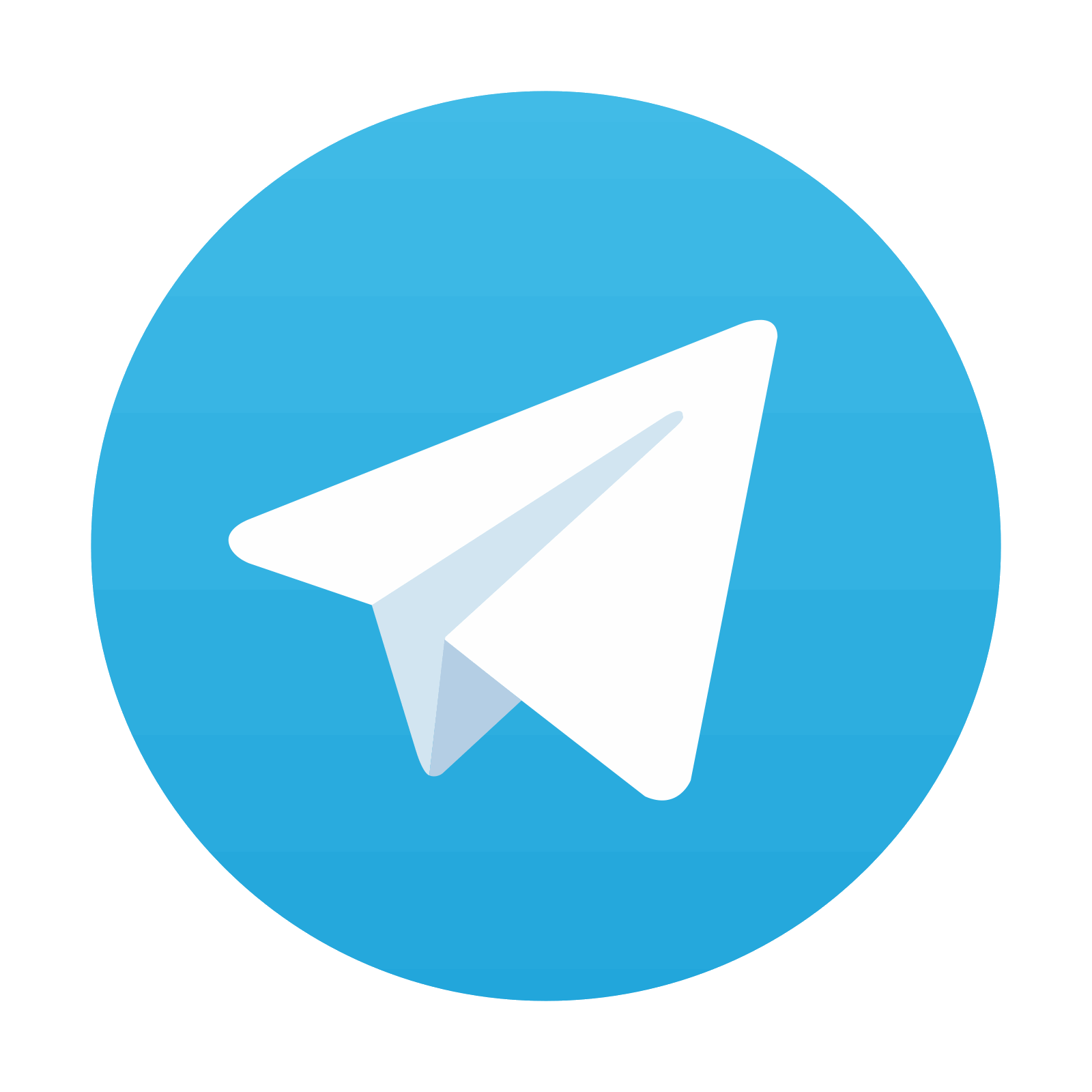
Stay updated, free articles. Join our Telegram channel
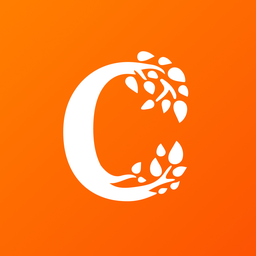
Full access? Get Clinical Tree
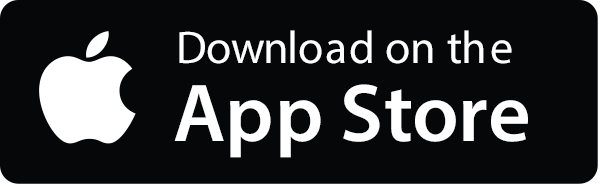
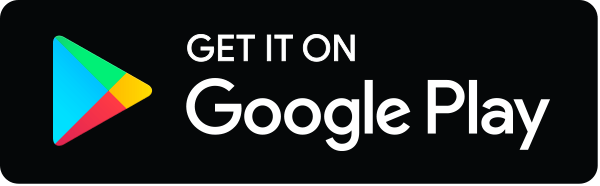